- Reference Manager
- Simple TEXT file
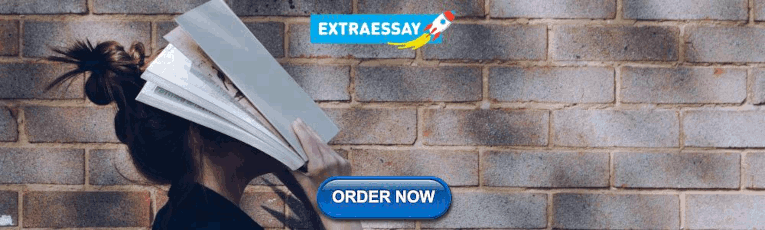
People also looked at
Mini review article, escherichia coli as a multifaceted pathogenic and versatile bacterium.

- Department of Biological Sciences, School of Pharmaceutical Sciences, São Paulo State University (UNESP), Araraquara, Brazil
Genetic plasticity promotes evolution and a vast diversity in Escherichia coli varying from avirulent to highly pathogenic strains, including the emergence of virulent hybrid microorganism. This ability also contributes to the emergence of antimicrobial resistance. These hybrid pathogenic E. coli (HyPEC) are emergent threats, such as O104:H4 from the European outbreak in 2011, aggregative adherent bacteria with the potent Shiga-toxin. Here, we briefly revisited the details of these E. coli classic and hybrid pathogens, the increase in antimicrobial resistance in the context of a genetically empowered multifaceted and versatile bug and the growing need to advance alternative therapies to fight these infections.
Introduction
Escherichia coli (or E. coli ) is a Gram-negative versatile bacterium, easily found and amenable to natural and random genetic alteration. There is a vast collection of sequenced E. coli genomes which exhibit different sizes and genomic diversity among commensal and pathogens, indicating a great assortment within the same bacterial species. They comprise of non-pathogenic bacteria that may act as commensals and belong to the normal intestinal microbiota of humans and many animals. There are also pathogenic variants, divided as diarrheagenic and extraintestinal pathogens, with different pathotypes and various natural hybrid strains ( Tables 1 and 2 ). These variants can be facultative or obligate pathogens. The facultative bacteria are part of the intestinal tract and may act as opportunistic pathogens when outside of their natural habitat, causing various types of extraintestinal infections. On the other hand, intestinal obligate pathogenic variants cause infections in distinct conditions, from moderate diarrhea to more threatening cases, as lethal outcome ( Kaper et al., 2004 ; Köhler and Dobrindt, 2011 ).
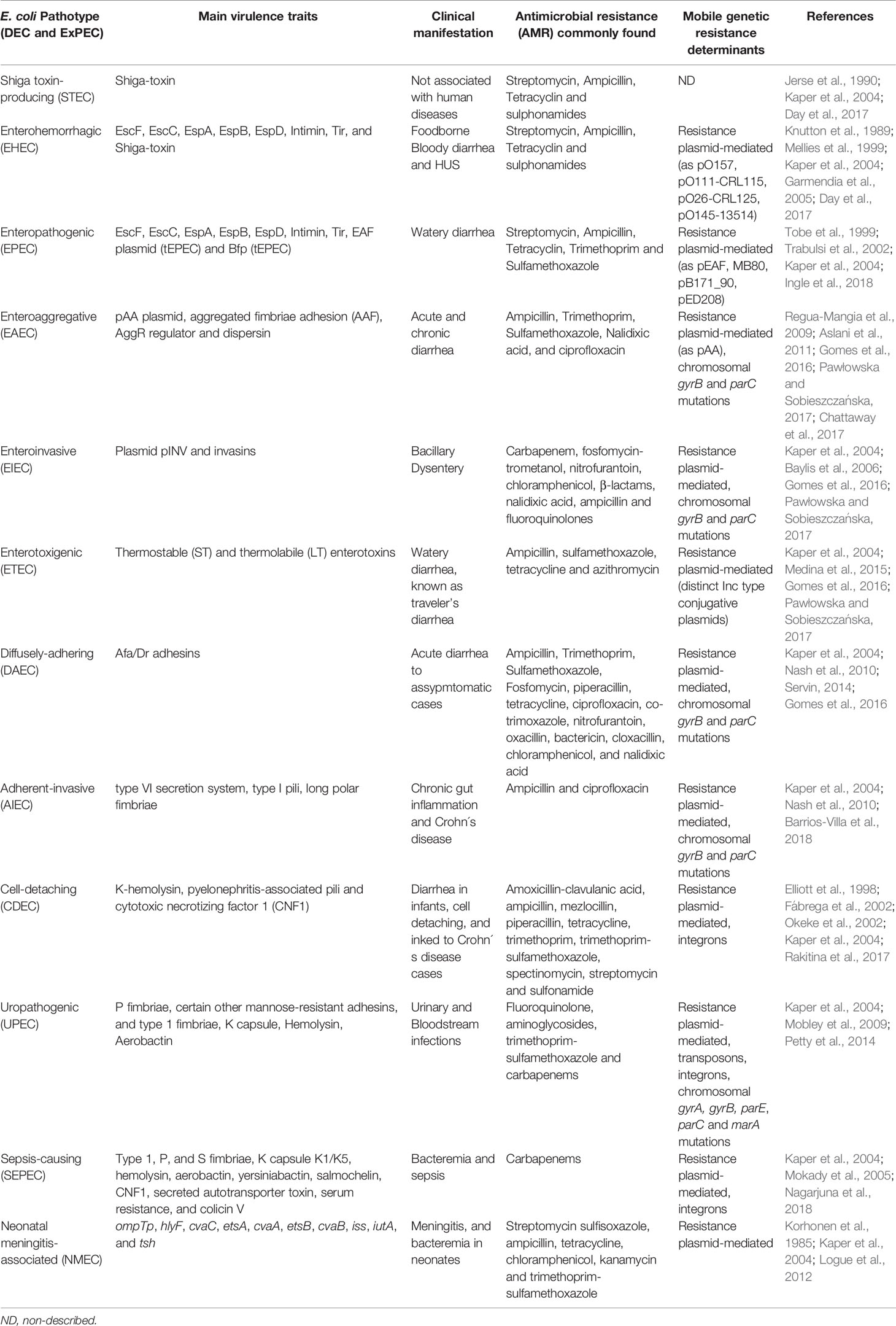
Table 1 Classic E. coli pathotypes main features: extraintestinal (ExPEC) and diarrheagenic (DEC).
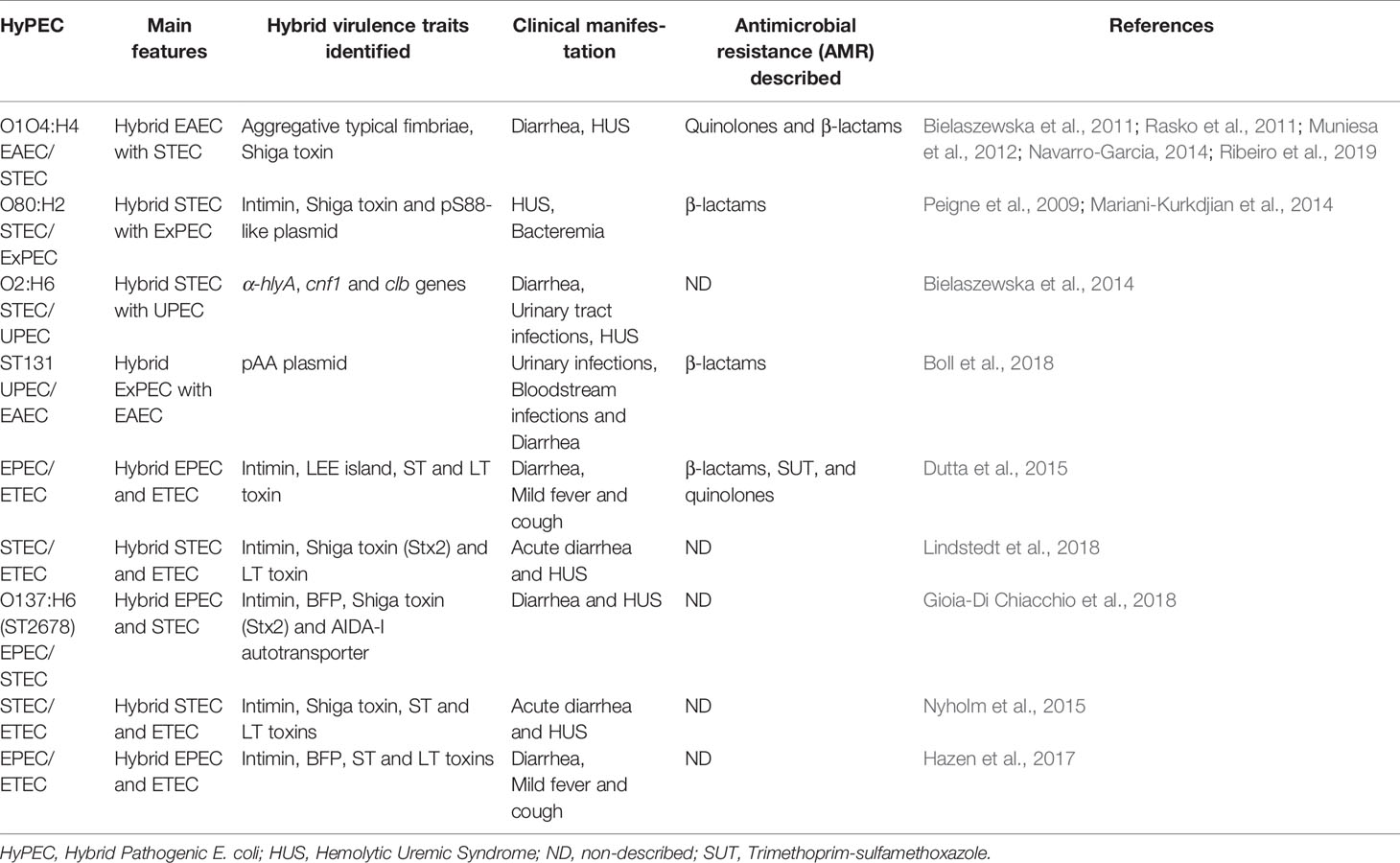
Table 2 Hybrid pathogenic (HyPEC) main features described.
E. coli pangenome studies indicate enormous capacity to evolve by gene acquisition and genetic modification. Besides, these genomes have a mosaic-like structure consisting of a core genome, encoding essential cellular functions, and an accessory genome with flexible strain-specific sequences. Thus, E. coli is a model well established for studying the interdependence of genome architecture and the lifestyle of bacteria ( Touchon et al., 2009 ; Dobrindt et al., 2010 ).
Based on virulence factors in E. coli genomes and phenotypic traits, the human pathotypes of diarrheagenic E. coli (DEC) are differentiated from non-pathogenic E. coli and extraintestinal pathogenic E. coli (ExPEC). The ExPEC are classified as uropathogenic E. coli (UPEC), sepsis-causing E. coli (SEPEC) and neonatal meningitis-associated E. coli (NMEC) ( Kaper et al., 2004 ). Recent pathogenomics and phenotypic classification have revisited the DEC group as nine distinct pathotypes, proposed by their differential features and the essential virulence genes defining each subgroup, such as Shiga toxin-producing E. coli (STEC), enterohemorrhagic E. coli (EHEC), enteropathogenic E. coli (EPEC), enterotoxigenic E. coli (ETEC), enteroinvasive E. coli (EIEC), enteroaggregative E. coli (EAEC), diffusely-adhering E. coli (DAEC), adherent-invasive E. coli (AIEC), and cell-detaching E. coli (CDEC) ( Kaper et al., 2004 ; Pawłowska and Sobieszczańska, 2017 ) ( Table 1 ).
Herein, we briefly describe the diversity of these classic and novel emerging E. coli pathotypes and their genetic plasticity in a multifaceted organism. The mobile genetic elements are responsible for the appearance of novel hybrid strains with distinct assortment of virulence and antimicrobial resistance traits, bringing up the urgent need to reconsider the forms of treatment for these infections.
Types of E. coli : Many Flavors Within a Single Bacterial Species
E. coli is one of the most genetically versatile microorganisms and is able to colonize and persist in several niches, both in the environment or in hosts. Commensal E. coli strains colonize the gastrointestinal tract of humans a few hours after birth, resulting in a symbiotic relationship between the microbiota and its host ( Ducarmon et al., 2019 ). However, the mechanisms by which E. coli ensures this efficient symbiosis is not well known. It could be related to its high ability to use nutrients in the colon ( Fabich et al., 2008 ; Ducarmon et al., 2019 ). Several studies have shown that competition for nutrients between microbiota and pathogens limits the colonization of the pathogens, leading to fierce competition among these microorganisms ( Lustri et al., 2017 ).
Occasionally, pathogenic E. coli cannot be distinguished from commensal E. coli , only based on specific virulence factors, as some previously described in ExPEC strains ( Köhler and Dobrindt, 2011 ). However, this scenario is changing due to sophistication and availability of molecular typing methodologies. New computational approaches bring countless important information about host-pathogen relationships, reservoir, clinical diagnoses, and novel ExPEC transmission pathways ( Johnson and Russo, 2018 ). Often, virulence genes are located in transmissible genetic elements such as genomic islands, bacteriophages, insertion sequences (ISs), integrons, plasmids, and transposons; hence, they can be easily exchanged among different bacteria ( Hacker et al., 2003 ; Dobrindt et al., 2010 ). They also carry multiple antibiotic resistance genes that have been under strong selective pressure as consequence of the extensive use of antibiotics ( Brzuszkiewicz et al., 2009 ).
Common genetic changes in E. coli genomes ensure high diversity due to the gain and loss of genes through genetic modification events. There are many strains of ExPEC that normally colonize the gut asymptomatically, as members of the intestinal microbiota. Nonetheless, only a subset of ExPEC as UPEC, SEPEC and NMEC are responsible for the vast majority of infections such as urinary tract infections, sepsis, and meningitis ( Kaper et al., 2004 ). There is a great variety of virulence factors in ExPEC strains, such as adhesins (fimbrial and non-fimbrial), siderophores, toxins, invasins, the ability to survive in serum, among others. Moreover, many of these virulence factors may occur combined within the same strain and act synergistically. Despite extra factors, the septic strains always possess at least an adherence system, an iron uptake system and genes for serum survival ( Biran and Ron, 2018 ; Johnson and Russo, 2018 ) ( Table 1 ).
The genetic evolution in E. coli pathogenesis employs horizontal transfer mechanisms within same and across similar species. Therefore, the IS, transposons and integrons may facilitate novel rearrangements within the genome, such as duplication and suppression of genes and also capture of new genes. This genetic material transit can result in greater flexibility concerning various features, such as the transition of pathogenic bacteria between humans and animals, resistance to antimicrobials, appearance of emerging pathogens due to the gain of virulence genes, increased pathogenicity, among other features ( Frost et al., 2005 ; Brigulla and Wackernagel, 2010 ; Dobrindt et al., 2010 ; Jackson et al., 2011 ; Sheppard et al., 2018 ). All these conditions may contribute to the virulence of these bacteria, like the bacteriophage importance in the pathogenesis. The horizontal transfer between different strains favors the emergence of new pathogenic strains with discrepancies in the bacteriophage repertoire affecting directly their virulence ( Manning et al., 2008 ; Ogura et al., 2009 ; Dobrindt et al., 2010 ; Jackson et al., 2011 ).
The co-evolution of bacterial genomes with plasmids, besides potential genetic and phenotypic gain may impact cellular metabolism to ensure the maintenance and stability of the plasmid ( Jackson et al., 2011 ). Many ExPEC virulence genes are encoded within plasmids, often belonging to the ColV family, which encodes colicin, serum survival factors and iron uptake systems ( Biran and Ron, 2018 ). Similarly, intestinal pathogens carry a variety of types of plasmids, associated with virulence, majorly belonging to the incompatibility group IncF, which has transfer functions ( Carattoli, 2009 ). There are virulence plasmids essential for some pathotypes of E. coli , such as pINV and pAA, respectively, in EIEC and EAEC, according to each own group features ( Kaper et al., 2004 ).
Although, all ExPEC and DEC pathotypes are not enough to fully classify all pathogenic E. coli strains, since these bacteria are so variable, allowing constant appearance of distinct hybrid-formed strains within this dynamic bacterial species. The carriage of virulence genes essential to the pathogenesis of each pathotype and the ability to adapt to different conditions allow the emergence of hybrid pathogenic E. coli (HyPEC).
Genetic Plasticity and Emergent E. coli Pathogen: HyPEC
E. coli has an astonishing facility to amend very well, replicate and disseminate. These features allowed the advent of novel HyPEC. Acquired virulence genes and novel functions appear from mutation, recombination and other genetic changes. All these genetic differences have increased the occurrence of novel hybrid and antimicrobial resistance among DEC and ExPEC ( Dobrindt et al., 2003 ; Bielaszewska et al., 2007 ; Khan et al., 2018 ).
Recently, a HyPEC strain received widespread attention after an outbreak of foodborne bloody diarrhea and hemorrhagic uremic syndrome (HUS) in Germany. This outbreak of E. coli O1O4:H4 was associated with consumption of raw fenugreek sprouts, as a hybrid EAEC strain with STEC features, like Shiga toxin presence. This HyPEC was quickly sequenced and unraveled its intricate nature, but even with a quick response and identification it was not enough to avoid 3,842 hospitalizations with many fatalities in Europe and North Africa ( Bielaszewska et al., 2011 ; Rasko et al., 2011 ). Emerging processes are responsible for the HyPEC occurrences. Herein, the combined enteroaggregative features in a rare serotype was responsible to high attachment to cells and a biofilm formation ( Navarro-Garcia, 2014 ; Ribeiro et al., 2019 ). Moreover, this strain has gained stx2 gene lambdoid phage integrated in the genome, thus it may release the Shiga-toxin. These features have increased HUS occurrence during the outbreak on this HyPEC when compared to STEC ( Muniesa et al., 2012 ).
Many distinct genetic hybrid examples are reported in E. coli , such as STEC/ExPEC O80:H2 serotype, which caused HUS and bacteremia due the presence of stx2 and eae genes from STECs and pS88-like plasmid, described in meningitis, urosepsis and avian pathogenic strains of ExPEC ( Peigne et al., 2009 ; Mariani-Kurkdjian et al., 2014 ). The STEC/UPEC strain O2:H6 serotype, a STEC with virulence genes as α-hlyA , cnf1 , and clb from UPEC that have ability to cause diarrhea and urinary tract infections ( Bielaszewska et al., 2014 ). The EPEC/ETEC strain has acquired the LEE island and encodes the LT toxin ( Dutta et al., 2015 ). The broadly reported multidrug resistant E. coli ST131 is example of highly virulent ExPEC associated with urinary and bloodstream infections. It has also acquired enteroaggregative diarrheagenic phenotype due to pAA plasmid presence ( Boll et al., 2018 ). Many others HyPEC are described as case report, but not fully characterized. Here, we have briefly sampled some of the acquired genes by these strains, their direct impact in virulence and their hybrid nature ( Table 2 ). Comparable to these HyPEC, the coined terms hybrid- and hetero-pathogenic E. coli have been recently described as new combination of virulence factors among classic E. coli groups. Together, they show differences between typical and atypical subgroups within the EAEC and EPEC pathotypes and hybrids, such as EPEC/STEC, ExPEC/EPEC and ExPEC/EAEC hybrids ( Santos et al., 2020 ). Similar to our approach here, this study shows how this topic is critical in the field.
The high prevalence of classic pathogenic E. coli and appearance of HyPEC occur via similar genetic mechanisms, which also enable bacteria to resist the presence of distinct antimicrobials. Bacteria resistant to various classes of antibiotics are related to the complex combination of intrinsic and acquired resistance genes, which may act synergistically ( Cag et al., 2016 ; Khan et al., 2018 ). Together that brings multiresistant bacteria, as an alarming factor reported worldwide in several bacterial species. WHO has prioritized studies on AMR bacteria, including Enterobacteriaceae, based on recent surveillance reports ( WHO, 2018 ).
Emerging Hybrids and Alternative Therapies
The complex combination of multidrug-resistant bacteria and emerging hybrid bacteria with intrinsic or acquired bacterial virulence factors disseminated by genetic mobility elements, the intense and inappropriate use of antibiotics have simultaneously favored the emergence of resistance to various antibiotics ( Khan et al., 2018 ). That is a special challenge to these hybrid strains, since these HyPEC gathered virulence traits and acquired antibiotic resistance, together these points raise the importance to alternative treatments. These options are crucial to reduce the use of antibiotics and the consequent increase of antimicrobial resistance. Novel therapies are urgent to replace prophylactic and treatment with antibiotics by probiotics, prebiotics, enzymatic compounds, vaccines, monoclonal antibodies, phage therapy, antivirulence compounds, among other possibilities ( Gadde et al., 2017 ).
Recently, different vaccine strategies have been used for pathogenic E. coli infection as an alternative to antibiotic therapy ( Rojas-Lopez et al., 2018 ), including vaccines with attenuated toxins ( McKenzie et al., 2007 ; Bitzan et al., 2009 ), attenuated bacterial cell ( Calderon Toledo et al., 2011 ), individual components of virulence factors such as Shiga toxin ( Liu et al., 2009 ), EspA or Intimin ( Oliveira et al., 2012 ), small peptides ( Zhang et al., 2011 ), DNA ( García-Angulo et al., 2014 ) or polysaccharides ( Ahmed et al., 2006 ; van den Dobbelsteen, 2016 ), as well detailed in the literature. Commercial vaccines have aimed the use to protect livestock, such as poultry, swine and bovine herds, against respectively to APEC, like Poulvac® E. coli , ETEC and EHEC infections ( Sadeyen et al., 2015 ; Nesta and Pizza, 2018 ). Vaccines with a modern approach and technology still are a promising strategy to protect against emergent HyPECs infections in humans and livestock.
Recent studies have revisited the phage therapy as a biological alternative, which employs strictly lytic phages uncapable of lysogenization ( Carter et al., 2012 ). Studies have demonstrated ability of phages to decrease biofilm formation in UPEC ( Chibeu et al., 2012 ), increased mice rate survival in E. coli -induced pneumonia ( Dufour et al., 2015 ). Moreover, lytic bacteriophages were used to infect and kill bacteria harboring phage-dependent conjugative plasmid to avoid emergence of multiresistant bacteria ( Ojala et al., 2013 ; Tagliaferri et al., 2019 ). The phages cocktail EcoShield™ is already commercialized (Intralytix) and it has been reported to significantly reduce the E. coli O157:H7 contamination on surfaces and food ( Abuladze et al., 2008 ; Carter et al., 2012 ). Additionally, mutual use of phages with antibiotics have emerged, with SPR02 and DAF6 phages combined with enrofloxacin have shown promising data, rescuing chickens challenged with avian pathogenic E. coli infection ( Tagliaferri et al., 2019 ).
The novel approach via antivirulence-directed compounds works disarming the pathogens’ ability to cause disease by inhibiting their virulence factors, favoring the host’s immune defenses during the bacterial clearance. These compounds do not induce bacterial resistance as antibiotics, because they disarm the pathogen, instead of directly targeting its growth. Therefore, as they are directed to specific factors for pathogenesis, they potentially reduce the selection of resistance and limit collateral damage to the microbiota. Some virulence inhibitors are effective against many pathogens, molecules such as LED209, HC102A, HC103A, Artemisinin, and Ethoxzolamide, by inhibit different two-component systems as QseBC in E. coli and other enteropathogens ( Sperandio et al., 2003 ; Rasko et al., 2008 ; Yang et al., 2014 ; Xue et al., 2015 ; Kim et al., 2020 ), Bicyclic 2-pyridones, Biaryl mannoside, Nitazoxanide and FN075, avoiding the initial bacterial adhesion; and compounds like Toxtazins A and B, Ebselen, 7086, 7812, 7832, BPT15, and BBH7, blocking toxins and secretion systems ( Payne, 2008 ; Johnson and Abramovitch, 2017 ).
The forces that shape the evolution in E. coli comprise vast repertoire, affecting genetic flexibility and excessive permissiveness to acquire and donate DNA via horizontal gene transfer. These features guarantee the spread of antibiotic resistance as well as virulence factors inherited among the various pathotypes of E. coli. The exact identification and assessment assist researchers to better understand this bacterium modification, diagnosis, public health and treatment. E. coli strains with multiple and distinct factors are probably very common but unreported, since these E. coli strains have developed many strategies to persist in different settings and successfully infect the host. These strategies result in an immense variety of microorganisms, ranging from avirulent to extremely virulent strains that can cause intestinal or extraintestinal diseases. E. coli strains have great potential for dissemination and capacity to pass along hereditary elements. Currently, these HyPEC strains are a very concerning threat that demands more studies and the development of novel treatment methods.
Author Contributions
VB: writing and organization. KM: writing. CM: writing and mentoring. All authors contributed to the article and approved the submitted version.
Financially supported by FAPESP (grants 2014/06779-2, 2018/22412-2, 2018/22042-0, and 2019/03049-7), CNPq (307418/2017-0), and “Programa de Apoio ao Desenvolvimento Científico da Faculdade de Ciências Farmacêuticas da UNESP-PADC. This study was financed in part by the Coordenação de Aperfeiçoamento de Pessoal de Nível Superior - Brasil (CAPES) - Finance Code 001.
Conflict of Interest
The authors declare that the research was conducted in the absence of any commercial or financial relationships that could be construed as a potential conflict of interest.
Abuladze T., Li M., Menetrez M. Y., Dean T., Senecal A., Sulakvelidze A. (2008). Bacteriophages reduce experimental contamination of hard surfaces, tomato, spinach, broccoli, and ground beef by Escherichia coli O157:H7. Appl. Environ. Microbiol. 74, 6230–62 8. doi: 10.1128/AEM.01465-08.21
PubMed Abstract | CrossRef Full Text | Google Scholar
Ahmed A., Li J., Shiloach Y., Robbins J. B., Szu S. C. (2006). Safety and immunogenicity of Escherichia coli O157 O-specific polysaccharide conjugate vaccine in 2-5-year-old children. J. Infect. Dis. 193, 515–521. doi: 10.1086/499821
Aslani M. M., Alikhani M. Y., Zavari A., Yousefi R., Zamani A. R. (2011). Characterization of enteroaggregative Escherichia coli (EAEC) clinical isolates and their antibiotic resistance pattern. Int. J. Infect. Dis. IJID Off. Publ. Intern. Soc Infec. Dis. 15 (2), e136–e139. doi: 10.1016/j.ijid.2010.10.002
CrossRef Full Text | Google Scholar
Barrios-Villa E., Cortés-Cortés G., Lozano-Zaraín P., Arenas-Hernández M., Martínez de la Peña C. F., Martínez-Laguna Y., et al. (2018). Adherent/invasive Escherichia coli (AIEC) isolates from asymptomatic people: new E. coli ST131 O25:H4/H30-Rx virotypes. Ann. Clin. Mic. Antim. 17 (1), 42. doi: 10.1186/s12941-018-0295-4
Baylis C. L., Penn C. W., Thielman N. M., Guerrant R. L., Jenkins C., Gillespie S. H. (2006). “Escherichia coli and Shigella spp,” in Principles and Practice of Clinical Bacteriology , 2nd ed. Eds. Gillespie S. H., Hawkey P. M. (England, UK: John Wiley and Sons Ltd), 347–365. doi: 10.1002/9780470017968.ch28
Bielaszewska M., Dobrindt U., Gärtner J., Gallitz I., Hacker J., Karch J., et al. (2007). Aspects of genome plasticity in pathogenic Escherichia coli . Int. J. Med. Microbiol. 297 (7-8), 625–639. doi: 10.1016/j.ijmm.2007.03.001
Bielaszewska M., Mellmann A., Zhang W., Köck R., Fruth A., Bauwens A., et al. (2011). Characterisation of the Escherichia coli strain associated with an outbreak of haemolytic uraemic syndrome in German: a microbiological study. Lancet Infect. Dis. 11 (9), 671–676. doi: 10.1016/S1473-3099(11)70165-7
Bielaszewska M., Schiller R., Lammers L., Bauwens A., Fruth A., Middendorf B., et al. (2014). Heteropathogenic virulence and phylogeny reveal phased pathogenic metamorphosis in Escherichia coli O2:H6. EMBO Mol. Med. 6, 347–357. doi: 10.1002/emmm.201303133
Biran D., Ron E. Z. (2018). Extraintestinal Pathogenic Escherichia coli . Curr. Top. Microbiol. Immunol. 416, 149–161. doi: 10.1007/82_2018_108
Bitzan M., Poole R., Mehran M., Sicard E., Brockus C., Thuning-Roberson C., et al. (2009). Safety and pharmacokinetics of chimeric anti-Shiga toxin 1 and anti-Shiga toxin 2 monoclonal antibodies in healthy volunteers. Antimicrob. Agents Chemother. 53, 3081–3087. doi: 10.1128/AAC.01661-08
Boll E. J., Overballe-Petersen S., Hasman H., Roer L., Ng K., Scheutz F., et al. (2018). Emergence of enteroaggregative Escherichia coli within the ST131 lineage as a cause of extraintestinal infections. mBio 11 (3), e00353–e00420. doi: 10.1128/mBio.00353-20
Brigulla M., Wackernagel W. (2010). Molecular aspects of gene transfer and foreign DNA acquisition in prokaryotes with regard to safety issues. Appl. Microbiol. Biotechnol. 86 (4), 1027–1041. doi: 10.1007/s00253-010-2489-3
Brzuszkiewicz E., Gottschalk G., Ron E., Hacker J., Dobrindt U. (2009). Adaptation of Pathogenic E. coli to Various Niches: Genome Flexibility is the Key. Genome Dyn. 6, 110–125. doi: 10.1159/000235766
Cag Y., Caskurlu H., Fan Y., Cao B., Vahaboglu H. (2016). Resistance mechanisms. Ann. Transl. Med. 4 (17), 326. doi: 10.21037/atm.2016.09.14
Calderon Toledo C., Arvidsson I., Karpman D. (2011). Cross-reactive protection against enterohemorrhagic Escherichia coli infection by enteropathogenic E. coli in a mouse model. Infect. Immun. 79, 2224–2233. doi: 10.1128/IAI.01024-10
Carattoli A. (2009). Resistance plasmid families in Enterobacteriaceae. Ant. Agents Chemother. 53 (6), 2227–2238. doi: 10.1128/AAC.01707-08
Carattoli A. (2013). Plasmids and the spread of resistance. Int. J. Med. Microbiol. 303, 298–304. doi: 10.1016/j.ijmm.2013.02.001
Carter C. D., Parks A., Abuladze T., Li M., Woolston J., Magnone J., et al. (2012). Bacteriophage cocktail significantly reduces Escherichia coli O157: H7 contamination of lettuce and beef, but does not protect against recontamination. Bacteriophage 2 (3), 178–185. doi: 10.4161/bact.22825
Chahales P., Hoffman P. S., Thanassi D. G. (2016). Nitazoxanide Inhibits Pilus Biogenesis by Interfering with Folding of the Usher Protein in the Outer Membrane. Antimicrob. Agents Chemother. 60, 2028–2038. doi: 10.1128/AAC.02221-15
Chattaway M. A., Day M., Mtwale J., White E., Rogers J., Day M., et al. (2017). Clonality, virulence and antimicrobial resistance of enteroaggregative Escherichia coli from Mirzapur, Bangladesh. J. Med. Microbiol. 66 (10), 1429–1435. doi: 10.1099/jmm.0.000594
Chibeu A., Lingohr E. J., Masson L., Manges A., Harel J., Ackermann H.-W., et al. (2012). Bacteriophages with the ability to degrade uropathogenic Escherichia coli biofilms. Viruses 4, 471–487. doi: 10.3390/v4040471
Curtis M. M., Russell R., Moreira C. G., Adebesin A. M., Wang C., Williams N. S., et al. (2014). QseC inhibitors as an antivirulence approach for Gram-negative pathogens. MBio 5 (6), e02165. doi: 10.1128/mBio.02165-14
Day M., Doumith M., Jenkins C., Dallman T. J., Hopkins K. L., Elson R., et al. (2017). Antimicrobial resistance in Shiga toxin-producing Escherichia coli serogroups O157 and O26 isolated from human cases of diarrhoeal disease in Englan. J. Antimicrob. Chemother. 72 (1), 145–152. doi: 10.1093/jac/dkw371
Dobrindt U., Agerer F., Michaelis K., Janka A., Buchrieser C., Samuelson M., et al. (2003). Analysis of genome plasticity in pathogenic and commensal Escherichia coli isolates by use of DNA arrays. J. Bacteriol. 185 (6), 1831–1840. doi: 10.1128/jb.185.6.1831-1840.2003
Dobrindt U., Chowdary M. G., Krumbholz G., Hacker J. (2010). Genome dynamics and its impact on evolution of Escherichia coli . Med. Microbiol. Immunol. 199 (3), 145–154. doi: 10.1007/s00430-010-0161-2
Ducarmon Q. R., Zwittink R. D., Hornung B. V. H., van Schaik W., Young V. B., Kuijper E. J. (2019). Gut Microbiota and Colonization Resistance against Bacterial Enteric Infection. Microbiol. Mol. Biol. Rev. 83 (3), e00007–e00019. doi: 10.1128/MMBR.00007-19
Dufour N., Debarbieux L., Fromentin M., Ricard J. D. (2015). Treatment of highly virulent extraintestinal pathogenic Escherichia coli pneumonia with bacteriophages. Crit. Care Med. 43 (6), e190–e198. doi: 10.1097/CCM.0000000000000968
Dutta S., Pazhani G. P., Nataro J. P., Ramamurthy T. (2015). Heterogenic virulence in a diarrheagenic Escherichia coli : evidence for an EPEC expressing heat-labile toxin of ETEC. Int. J. Med. Microbiol. 305, 47–54. doi: 10.1016/j.ijmm.2014.10.006
Elbediwi M., Li Y., Paudyal N., Pan H., Li X., Xie S., et al. (2019). Global Burden of Colistin-Resistant Bacteria: Mobilized Colistin Resistance Genes Study, (1980-2018). Microorganisms 7 (10), E461. doi: 10.3390/microorganisms7100461
Elliott S. J., Srinivas S., Albert M. J., Alam K., Robins-Browne R. M., Gunzburg S. T., et al. (1998). Characterization of the roles of hemolysin and other toxins in enteropathy caused by alpha-hemolytic Escherichia coli linked to human diarrhea. Infect. Immun. 66, 2040–2051. doi: 10.1128/IAI.66.5.2040-2051.1998
Fabich A. J., Jones S. A., Chowdhury F. Z., Cernosek A., Anderson A., Smalley D., et al. (2008). Comparison of carbon nutrition for pathogenic and commensal Escherichia coli strains in the mouse intestine. Infect. Immun. 76, 1143–1152. doi: 10.1128/IAI.01386-07
Fábrega V. L. A., Ferreira A. J. P., Patrício F. R. S., Brinkley C., Scaletsky I. C. A. (2002). Cell-detaching Escherichia coli (CDEC) strains from children with diarrhea: Identification of a protein with toxigenic activity. FEMS Microbiol. Lett. 217 (2), 191–197. doi: 10.1111/j.1574-6968.2002.tb11474.x
Fernandes M. R., McCulloch J. A., Vianello M. A., Moura Q., Perez-Chaparro P. J., Esposito F., et al. (2016). First Report of the Globally Disseminated IncX4 Plasmid Carrying the mcr-1 Gene in a Colistin-Resistant Escherichia coli Sequence Type 101 Isolate from a Human Infection in Brazil. Antimic. Agents Chemother. 60 (10), 6415–6417. doi: 10.1128/AAC.01325-16
Frost L. S., Leplae R., Summers A. O., Toussaint A. (2005). Mobile genetic elements: the agents of open source evolution. Nat. Rev. Microbiol. 3 (9), 722–732. doi: 10.1038/nrmicro1235
Gadde U., Kim W. H., Oh S. T., Lillehoj H. S. (2017). Alternatives to antibiotics for maximizing growth performance and feed efficiency in poultry: a review. Anim. Health Res. Rev. 18 (1), 26–45. doi: 10.1017/S1466252316000207
García-Angulo V. A., Kalita A., Kalita M., Lozano L., Torres A. G. (2014). Comparative genomics and immunoinformatics approach for the identification of vaccine candidates for enterohemorrhagic Escherichia coli O157:H7. Infect. Immun. 82, 2016–2026. doi: 10.1128/IAI.01437-13
Garmendia J., Frankel G., Crepin V. F. (2005). Enteropathogenic and enterohemorrhagic Escherichia coli infections: translocation, translocation, translocation. Infect. Immun. 73 (5), 2573–2585. doi: 10.1128/IAI.73.5.2573-2585.2005
Gioia-Di Chiacchio R. M., Cunha M. P. V., de Sá L. R. M., Davies Y. M., Pereira C. B. P., Martins F. H., et al. (2018). Novel Hybrid of Typical Enteropathogenic Escherichia coli and Shiga-Toxin-Producing E. coli (tEPEC/STEC) Emerging From Pet Birds. Front. Microbiol. 9:2975:2975. doi: 10.3389/fmicb.2018.02975
Gomes T. A., Elias W. P., Scaletsky I. C., Guth B. E., Rodrigues J. F., Piazza R. M. (2016). Diarrheagenic Escherichia coli . Braz. J. Microbiol. 47 Suppl 1, 3–30. doi: 10.1016/j.bjm.2016.10.015
Gunzburg S. T., Chang B. J., Elliott S. J., Burke V., Gracey M. (1993). Diffuse and enteroaggregative patterns of adherence of enteric Escherichia coli isolated from aboriginal children from the Kimberley region of Western Australia. J. Infect. Dis. 167, 755–758. doi: 10.1093/infdis/167.3.755
Hacker J., Hentschel U., Dobrindt U. (2003). Prokaryotic chromosomes and disease. Science 301 (5634), 790–793. doi: 10.1126/science.1086802
Hadjifrangiskou M., Kostakioti M., Chen S. L., Henderson J. P., Greene S. E., Hultgren S. J. (2011). A central metabolic circuit controlled by QseC in pathogenic Escherichia coli . Mol. Microbiol. 80 (6), 1516–1529. doi: 10.1111/j.1365-2958.2011.07660.x
Han Z., Pinkner J. S., Ford B., Chorell E., Crowley J. M., Cusumano C. K., et al. (2012). Lead optimization studies on FimH antagonists: discovery of potent and orally bioavailable ortho-substituted biphenyl mannosides. J. Med. Chem. 55, 3945–3959. doi: 10.1021/jm300165m
Hazen T. H., Michalski J., Luo Q., Shetty A. C., Daugherty S. C., Fleckenstein J. M., et al. (2017). Comparative genomics and transcriptomics of Escherichia coli isolates carrying virulence factors of both enteropathogenic and enterotoxigenic E. coli . Sci. Rep. 7, 3513. doi: 10.1038/s41598-017-03489-z
Ingle D. J., Levine M. M., Kotloff K. L., Holt K. E., Robins-Browne R. M. (2018). Dynamics of antimicrobial resistance in intestinal Escherichia coli from children in community settings in South Asia and sub-Saharan Africa. Nat. Microbiol. 3 (9), 1063–1073. doi: 10.1038/s41564-018-0217-4
Jackson R. W., Vinatzer B., Arnold D. L., Dorus S., Murillo J. (2011). The influence of the accessory genome on bacterial pathogen evolution. Mob. Genet. Elements 1 (1), 55–65. doi: 10.4161/mge.1.1.16432
Jarvis C., Han Z., Kalas V., Klein R., Pinkner J. S., Ford B., et al. (2016). Antivirulence Isoquinolone Mannosides: Optimization of the Biaryl Aglycone for FimH Lectin Binding Affinity and Efficacy in the Treatment of Chronic UTI. ChemMedChem 11 (4), 367–373. doi: 10.1002/cmdc.201600006
Jerse A. E., Yu J., Tall B. D., Kaper J. B. (1990). A genetic locus of enteropathogenic Escherichia coli necessary for the production of attaching and effacing lesions on tissue culture cells. Proc. Natl. Acad. Sci. U.S.A. 87 (20), 7839–7843. doi: 10.1073/pnas.87.20.7839
Johnson B. K., Abramovitch R. B. (2017). Small Molecules That Sabotage Bacterial Virulence. Trends Pharmacol. Sci. 38 (4), 339–362. doi: 10.1016/j.tips.2017.01.004
Johnson J. R., Russo T. A. (2018). Molecular Epidemiology of Extraintestinal Pathogenic Escherichia coli . EcoSal. Plus 8 (1), 4–22. doi: 10.1128/ecosalplus.ESP-0004-2017
Kaper J. B., Nataro J. P., Mobley H. L. (2004). Pathogenic Escherichia coli . Nat. Rev. Microbiol. 2 (2), 123–140. doi: 10.1038/nrmicro818
Khan A., Miller W. R., Arias C. A. (2018). Mechanisms of antimicrobial resistance among hospital-associated pathogens. Expert Rev. Anti. Infect. Ther. 16 (4), 269–287. doi: 10.1080/14787210.2018.1456919
Kim C. S., Gatsios A., Cuesta S., Lam Y. C., Wei Z., Chen H., et al. (2020). Characterization of Autoinducer-3 Structure and Biosynthesis in E. coli . ACS Cent. Sci. 6 (2), 197–206. doi: 10.1021/acscentsci.9b01076
Knutton S., Baldwin T., Williams P. H., McNeish A. S. (1989). Actin accumulation at sites of bacterial adhesion to tissue culture cells: basis of a new diagnostic test for enteropathogenic and enterohemorrhagic Escherichia coli . Infect. Immun. 57 (4), 1290–1298. doi: 10.1128/IAI.57.4.1290-1298.1989
Köhler C. D., Dobrindt U. (2011). What defines extraintestinal pathogenic Escherichia coli ? Int. J. Med. Microbiol. 301 (8), 642–647. doi: 10.1016/j.ijmm.2011.09.006
Korhonen T. K., Valtonen M. V., Parkkinen J., Väisänen-Rhen V., Finne J., Orskov F., et al. (1985). Serotypes, hemolysin production, and receptor recognition of Escherichia coli strains associated with neonatal sepsis and meningitis. Infect. Immun. 48 (2), 486–491. doi: 10.1128/IAI.48.2.486-491.1985
Lindstedt B. A., Finton M. D., Porcellato D., Brandal L. T. (2018). High frequency of hybrid Escherichia coli strains with combined Intestinal Pathogenic Escherichia coli (IPEC) and Extraintestinal Pathogenic Escherichia coli (ExPEC) virulence factors isolated from human faecal samples. BMC Infect. Dis. 18, 544. doi: 10.1186/s12879-018-3449-2
Liu J., Sun Y., Feng S., Zhu L., Guo X., Qi C. (2009). Towards an attenuated enterohemorrhagic Escherichia coli O157:H7 vaccine characterized by a deleted ler gene and containing apathogenic Shiga toxins. Vaccine 27, 5929–5935. doi: 10.1016/j.vaccine.2009.07.097
Logue C. M., Doetkott C., Mangiamele P., Wannemuehler Y. M., Johnson T. J., Tivendale K. A., et al. (2012). Genotypic and phenotypic traits that distinguish neonatal meningitis-associated Escherichia coli from fecal E. coli isolates of healthy human hosts. Appl. Environ. Microbiol. 78 (16), 5824–5830. doi: 10.1128/AEM.07869-11
Lustri B. C., Sperandio V., Moreira C. G. (2017). Bacterial chat: intestinal metabolites and signals in host-microbiota-pathogen interactions. Infec. Immun. 85 (12), e00476. doi: 10.1128/IAI.00476-17
Maltby R., Leatham-Jensen M. P., Gibson T., Cohen P. S., Conway T. (2013). Nutritional basis for colonization resistance by human commensal Escherichia coli strains HS and Nissle 1917 against E. coli O157:H7 in the mouse intestine. PloS One 8, e53957. doi: 10.1371/journal.pone.0053957
Manning S. D., Motiwala A. S., Springman A. C., Qi W., Lacher D. W., Ouellette L. M., et al. (2008). Variation in virulence among clades of Escherichia col i O157:H7 associated with disease outbreaks. Proc. Natl. Acad. Sci. U.S.A. 105 (12), 4868–4873. doi: 10.1073/pnas.0710834105
Mariani-Kurkdjian P., Lemaitre C., Bidet P., Perez D., Boggini L., Kwon T., et al. (2014). Haemolytic-uraemic syndrome with bacteraemia caused by a new hybrid Escherichia coli pathotype. New Microbes New Infect. 2, 127–131. doi: 10.1002/nmi2.49
Marques L. R., Abe C. M., Grin P. M., Gomes T. A. T. (1995). Association between alpha-hemolysin production and HeLa cell-detaching activity in fecal isolates of Escherichia coli . J. Clin. Microbiol. 33, 2707–2709. doi: 10.1128/JCM.33.10.2707-2709.1995
McKenzie R., Bourgeois A. L., Frech S. A., Flyer D. C., Bloom A., Kazempour K., et al. (2007). Transcutaneous immunization with the heat-labile toxin (LT) of enterotoxigenic Escherichia coli (ETEC): protective efficacy in a double-blind, placebo-controlled challenge study. Vaccine 25, 3684–3691. doi: 10.1016/j.vaccine.2007.01.043
Medina A. M., Rivera F. P., Pons M. J., Riveros M., Gomes C., Bernal M., et al. (2015). Comparative analysis of antimicrobial resistance in enterotoxigenic Escherichia coli isolates from two paediatric cohort studies in Lima, Peru. Trans. R. Soc Trop. Med. Hyg. 109 (8), 493–502. doi: 10.1093/trstmh/trv054
Mellies J. L., Elliott S. J., Sperandio V., Donnenberg M. S., Kaper J. B. (1999). The Per regulon of enteropathogenic Escherichia coli : identification of a regulatory cascade and a novel transcriptional activator, the locus of enterocyte effacement (LEE)-encoded regulator (Ler). Mol. Microbiol. 33 (2), 296–306. doi: 10.1046/j.1365-2958.1999.01473.x
Mobley H., Donnenberg M., Hagan E. (2009). Uropathogenic Escherichia coli , EcoSal Plus 2009. EcoSal Plus 3 (2), 1–27. doi: 10.1128/ecosalplus.8.6.1.3
Mokady D., Gophna U., Ron E. Z. (2005). Virulence factors of septicemic Escherichia coli strains. Int. J. Med. Microbiol. 295 (6-7), 455–462. doi: 10.1016/j.ijmm.2005.07.007
Muniesa M., Hammerl J. A., Hertwig S., Appel B., Brüssow H. (2012). Shiga toxin-producing Escherichia coli O104: H4: a new challenge for microbiology. Appl. Environ. Microbiol. 78 (12), 4065–4073. doi: 10.1128/AEM.00217-12
Nagarjuna D., Mittal G., Dhanda R. S., Gaind R., Yadav M. (2018). Alarming levels of antimicrobial resistance among sepsis patients admitted to ICU in a tertiary care hospital in India - a case control retrospective study. Antimicrob. Resist. Infect. Control 7, 150. doi: 10.1186/s13756-018-0444-8
Nash J. H., Villegas A., Kropinski A. M., Aguilar-Valenzuela R., Konczy P., Mascarenhas M., et al. (2010). Genome sequence of adherent-invasive Escherichia coli and comparative genomic analysis with other E. coli pathotypes. BMC Genomics 11, 667. doi: 10.1186/1471-2164-11-667
Navarro-Garcia F. (2014). Escherichia coli O104:H4 Pathogenesis: an Enteroaggregative E.coli /Shiga Toxin-Producing E. coli Explosive Cocktail of HighVirulence. Microbiol. Spectr. 2 (6), 2–15. doi: 10.1128/microbiolspec.EHEC-0008-2013
Nesta B., Pizza M. (2018). “Vaccines against Escherichia coli.“ In Escherichia coli, a Versatile Pathogen (Cham: Springer), 213–242. doi: 10.1007/82_2018_111
Nyholm O., Halkilahti J., Wiklund G., Okeke U., Paulin L., Auvinen P., et al. (2015). Comparative genomics and characterization of hybrid Shigatoxigenic and Enterotoxigenic Escherichia coli (STEC/ETEC) strains. PloS One 10, e0135936. doi: 10.1371/journal.pone.0135936
Ogura Y., Ooka T., Iguchi A., Toh H., Asadulghani M., Oshima K., et al. (2009). Comparative genomics reveal the mechanism of the parallel evolution of O157 and non-O157 enterohemorrhagic Escherichia coli . Proc. Natl. Acad. Sci. U.S.A. 106 (42), 17939–17944. doi: 10.1073/pnas.0903585106
Ojala V., Laitalainen J., Jalasvuori M. (2013). Fight evolution with evolution: plasmid-dependent phages with a wide host range prevent the spread of antibiotic resistance. Evol. Appl. 6, 925–932. doi: 10.1111/eva.12076
Okeke I. N., Steinrück H., Kanack K. J., Elliott S. J., Sundström L., Kaper J. B., et al. (2002). Antibiotic-resistant cell-detaching Escherichia coli strains from Nigerian children. J. Clin. Microb. 40 (1), 301–305. doi: 10.1128/jcm.40.1.301-305.2002
Oliveira A. F., Cardoso S. A., Almeida F. B., de Oliveira L. L., Pitondo-Silva A., Soares S. G., et al. (2012). Oral immunization with attenuated Salmonella vaccine expressing Escherichia coli O157:H7 intimin gamma triggers both systemic and mucosal humoral immunity in mice. Microbiol. Immunol. 56, 513–522. doi: 10.1111/j.1348-0421.2012.00477.x
Pawłowska B., Sobieszczańska B. M. (2017). Intestinal epithelial barrier: The target for pathogenic Escherichia coli . Adv. Clin. Exp. Med. 26 (9), 1437–1445. doi: 10.17219/acem/64883
Payne D. J. (2008). Microbiology. Desperately seeking new antibiotics. Science 321 (5896), 1644–1645. doi: 10.1126/science.1164586
Peigne C., Bidet P., Mahjoub-Messai F., Plainvert C., Barbe V., Médigue C., et al. (2009). The plasmid of Escherichia coli strain S88 (O45:K1:H7) that causes neonatal meningitis is closely related to avian pathogenic E. coli plasmids and is associated with high-level bacteremia in a neonatal rat meningitis model. Infect. Immune 77 (6), 2272–2284. doi: 10.1128/IAI.01333-08
Petty N. K., Ben Zakour N. L., Stanton-Cook M., Skippington E., Totsika M., Forde B. M., et al. (2014). Global dissemination of a multidrug resistant Escherichia coli clone. Proc. Nat. Acad. Sci. U.S.A. 111 (15), 5694–5699. doi: 10.1073/pnas.1322678111
Pinkner J. S., Remaut H., Buelens F., Miller E., Aberg V., Pemberton N., et al. (2006). Rationally designed small compounds inhibit pilus biogenesis in uropathogenic bacteria. Proc. Natl. Acad. Sci. U.S.A. 103, 17897–17902. doi: 10.1073/pnas.0606795103
Rakitina D. V., Manolov A. I., Kanygina A. V., Garushyants S. K., Baikova J. P., Alexeev D. G., et al. (2017). Genome analysis of E. coli isolated from Crohn’s disease patients. BMC Genomics 18 (1), 544. doi: 10.1186/s12864-017-3917-x
Rasko D. A., Moreira C. G., de Li R., Reading N. C., Ritchie J. M., Waldor M. K., et al. (2008). Targeting QseC signaling and virulence for antibiotic development. Science 321 (5892), 1078–1080. doi: 10.1126/science.1160354
Rasko D. A., Webster D. R., Sahl J. W., Bashir A., Boisen N., Scheutz F., et al. (2011). Origins of the E. coli strain causing an outbreak of hemolytic-uremic syndrome in Germany. N Engl. J. Med. 365 (8), 709–717. doi: 10.1056/NEJMoa1106920
Regua-Mangia A. H., Gomes T. A., Vieira M. A., Irino K., Teixeira L. M. (2009). Molecular typing and virulence of enteroaggregative Escherichia coli strains isolated from children with and without diarrhoea in Rio de Janeiro city, Brazil. J. Med. Microbiol. 58, 414–422. doi: 10.1099/jmm.0.006502-0
Ribeiro T. R. M., Lustri B. C., Elias W. P., Moreira C. G. (2019). QseC Signaling in the Outbreak O104:H4 Escherichia coli Strain Combines Multiple Factors during Infection. J. Bacteriol. Aug. 8, e00203–e00219, 201(17). doi: 10.1128/JB.00203-19
Rodríguez-Martínez J. M., Machuca J., Cano M. E., Calvo J., Martínez-Martínez L., Pascual A. (2016). Plasmid-mediated quinolone resistance: two decades on. Drug Resist. Update 29, 13–29. doi: 10.1016/j.drup.2016.09.001
Rojas-Lopez M., Monterio R., Pizza M., Desvaux M., Rosini R. (2018). Intestinal pathogenic Escherichia coli : insights for vaccine development. Front. Microbiol. 9, 440. doi: 10.3389/fmicb.2018.00440
Rooks M. G., Veiga P., Reeves A. Z., Lavoie S., Yasuda K., Asano Y., et al. (2017). QseC inhibition as an antivirulence approach for colitis-associated bacteria. Proc. Natl. Acad. Sci. U.S.A. 114 (1), 142–147. doi: 10.1073/pnas.1612836114
Sadeyen J.-R., Wu Z., Davies H., van Diemen P. M., Milicic A., La Ragione R. M., et al. (2015). Immune responses associated with homologous protection conferred by commercial vaccines for control of avian pathogenic Escherichia coli in turkeys. Vet. Res. 461), 5. doi: 10.1186/s13567-014-0132-5
Santos A. C. M., Santos F. F., Silva R. M., Gomes T. A. T. (2020). Diversity of Hybrid- and Hetero-Pathogenic Escherichia coli and Their Potential Implication in More Severe Diseases. Front. Cell. Infect. Microbiol. 10, 339. doi: 10.3389/fcimb.2020.00339
Servin A. L. (2014). Pathogenesis of human diffusely adhering Escherichia coli expressing Afa/Dr adhesins (Afa/Dr DAEC): current insights and future challenges. Clin. Mic. Rev. 27 (4), 823–869. doi: 10.1128/CMR.00036-14
Shamir E. R., Warthan M., Brown S. P., Nataro J. P., Guerrant R. L., Hoffman P. S. (2010). Nitazoxanide inhibits biofilm production and hemagglutination by enteroaggregative Escherichia coli strains by blocking assembly of AafA fimbriae. Antimicrob. Agents Chemother. 54, 1526–1533. doi: 10.1128/AAC.01279-09
Sheppard S. K., Guttman D. S., Fitzgerald J. R. (2018). Population genomics of bacterial host adaptation. Nat. Rev. Genet. 19 (9), 549–565. doi: 10.1038/s41576-018-0032-z
Sperandio V., Torres A. G., Jarvis B., Nataro J. P., Kaper J. B. (2003). Bacteria-host communication: the language of hormones. PNAS 22100 (15), 8951–8956. doi: 10.1073/pnas.1537100100
Tagliaferri T. L., Mathias J., Hans-Peter H. (2019). Fighting pathogenic bacteria on two fronts: phages and antibiotics as combined strategy. Front. Cel. Infect. Microb. 9, 22. doi: 10.3389/fcimb.2019.00022
Tobe T., Hayashi T., Han C. G., Schoolnik G. K., Ohtsubo E., Sasakawa C. (1999). Complete DNA sequence and structural analysis of the enteropathogenic Escherichia coli adherence factor plasmid. Infect. Immun. 67 (10), 5455–5462. doi: 10.1128/IAI.67.10.5455-5462.1999
Touchon M., Hoede C., Tenaillon O., Barbe V., Baeriswyl S., Bidet P., et al. (2009). Organised genome dynamics in the Escherichia coli species results in highly diverse adaptive paths. PloS Genet. 5 (1), e1000344. doi: 10.1371/journal.pgen.1000344
Trabulsi L. R., Keller R., Tardelli Gomes T. A. (2002). Typical and atypical enteropathogenic Escherichia coli . Emerg. Infect. Dis. 8 (5), 508–513. doi: 10.3201/eid0805.010385
van den Dobbelsteen G., Fae K. C., Serroyen J., van den Nieuwenhof I. M., Braun M., Haeuptle M. A., et al. (2016). Immunogenicity and safety of a tetravalent E. coli O-antigen bioconjugate vaccine in animal models. Vaccine 34, 4152e60. doi: 10.1016/j.vaccine.2016.06.067
World Health Organization. (2018). Global antimicrobial resistance surveillance system (GLASS) report. Early implemetantion 2016-2017 , ISBN: ISBN: 978 92 4 151344-9.
Google Scholar
Xue X. Y., Mao X. G., Li Z., Chen Z., Zhou Y., Hou Z., et al. (2015). A potent and selective antimicrobial poly(amidoamine) dendrimer conjugate with LED209 targeting QseC receptor to inhibit the virulence genes of gram-negative bacteria. Nanomedicine 11 (2), 329–339. doi: 10.1016/j.nano.2014.09.016
Yang Q., Anh N. D., Bossier P., Defoirdt T. (2014). Norepinephrine and dopamine increase motility, biofilm formation, and virulence of Vibrio harveyi . Front. Microbiol. 5:584:584. doi: 10.3389/fmicb.2014.00584
Zhang X. H., He K. W., Zhang S. X., Lu W. C., Zhao P. D., Luan X. T., et al. (2011). Subcutaneous and intranasal immunization with Stx2B-Tir-Stx1B-Zot reduces colonization and shedding of Escherichia coli O157:H7 in mice. Vaccine 29, 3923–3929. doi: 10.1016/j.vaccine.2011.02.007
Zheng B., Dong H., Xu H., Lv J., Zhang J., Jiang X., et al. (2016). Coexistence of MCR-1 and NDM-1 in Clinical Escherichia coli Isolates. Clin. Infect. Dis. 63 (10), 1393–1395. doi: 10.1093/cid/ciw553
Zhong L. L., Zhang Y. F., Doi Y., Huang X., Zhang X. F., Zeng K. J., et al. (2017). Coproduction of MCR-1 and NDM-1 by Colistin-Resistant Escherichia coli Isolated from a Healthy Individual. Antimicrob. Agents Chemother. 61 (1), e01962–e01916. doi: 10.1128/AAC.01962-16
Keywords: treatment, genetic mobility, pathogenesis, Escherichia , multiresistant
Citation: Braz VS, Melchior K and Moreira CG (2020) Escherichia coli as a Multifaceted Pathogenic and Versatile Bacterium. Front. Cell. Infect. Microbiol. 10:548492. doi: 10.3389/fcimb.2020.548492
Received: 02 April 2020; Accepted: 17 November 2020; Published: 21 December 2020.
Reviewed by:
Copyright © 2020 Braz, Melchior and Moreira. This is an open-access article distributed under the terms of the Creative Commons Attribution License (CC BY) . The use, distribution or reproduction in other forums is permitted, provided the original author(s) and the copyright owner(s) are credited and that the original publication in this journal is cited, in accordance with accepted academic practice. No use, distribution or reproduction is permitted which does not comply with these terms.
*Correspondence: Cristiano Gallina Moreira, [email protected]
† ORCID : Vânia Santos Braz, orcid.org/0000-0002-1389-1055 Cristiano Gallina Moreira, orcid.org/0000-0002-0689-4119
This article is part of the Research Topic
Interaction of Pathogenic Escherichia coli with the Host: Pathogenomics, Virulence and Antibiotic Resistance

An official website of the United States government
The .gov means it’s official. Federal government websites often end in .gov or .mil. Before sharing sensitive information, make sure you’re on a federal government site.
The site is secure. The https:// ensures that you are connecting to the official website and that any information you provide is encrypted and transmitted securely.
- Publications
- Account settings
Preview improvements coming to the PMC website in October 2024. Learn More or Try it out now .
- Advanced Search
- Journal List
- Life (Basel)

Horizontal Gene Transfer among Bacteria and Its Role in Biological Evolution
Associated data.
This is a contribution to the history of scientific advance in the past 70 years concerning the identification of genetic information, its molecular structure, the identification of its functions and the molecular mechanisms of its evolution. Particular attention is thereby given to horizontal gene transfer among microorganisms, as well as to biosafety considerations with regard to beneficial applications of acquired scientific knowledge.
1. Introduction
The discovery of horizontal gene transfer is related to the introduction of experimental microbial genetics some 70 years ago. Soon thereafter, medical microbiology identified the raising problem of increasing antibiotic resistance of pathogenic bacteria. This medical problem stimulated research in bacterial genetics which revealed that horizontal gene transfer is involved in some of the genetic variations causing resistance to antibiotics.
As a contribution to the history of scientific investigations, we trace here a sequence of steps of conceptual and experimental approaches to understand microbial evolution at the molecular level. This shall allow us to extrapolate to generally valid laws of nature guiding biological evolution by self-organization. We will also discuss implications of the acquired scientific knowledge.
2. Roots of Microbial Genetics
It is in the first half of the 20th century that microbiologists became aware that bacterial isolates and bacterial viruses (bacteriophages) under study could spontaneously produce phenotypic variants. This property offered the chance to investigate recombination between different mutants and between different microbial strains, i.e. , to carry out genetic experiments with bacteria as well as with bacteriophages.
2.1. The Discovery of Bacterial Transformation
Early work with pneumococcal bacteria has identified two forms: smooth (S) cells which are virulent, and rough (R) cells which are not virulent as seen upon infection of mice in laboratory tests. Using this experimental system, Griffith [ 1 ] infected mice both with living R variants and with heat-killed S bacteria. After some time, many of the doubly infected mice developed pneumonia and in their blood S type bacteria were found. This indicated that recombination had occurred between the living R form and a substance of the heat-killed S form bacteria. In the subsequent search for the identity of the substance causing virulence, Avery, MacLeod and McCarty [ 2 ] identified a highly purified DNA fraction to bring about recombinants, whereas no other fraction of the heat-killed S bacteria caused recombination. This rather unexpected result clearly indicated that DNA is the carrier of genetic information. A majority of biologists hesitated to accept this novel knowledge since one had expected that the highly complex and specific genetic information would rather be carried on more complex molecules than DNA, e.g., by proteins. This problem found its solution almost ten years later when Hershey and Chase [ 3 ] showed that bacteriophage T2 injects its DNA, but not proteins upon infection of host bacteria, and when the double-helical filamentous structure of DNA molecules was described by Watson and Crick [ 4 ]. These authors proposed genetic information to be contained in the specific sequences of nucleotides.
2.2. Bacterial Conjugation
In the same time period, several researchers also tested the possibility of recombination between bacterial isolates with different phenotypes upon mixed incubation. This happened to occur in some of the mixtures, in particular those involving phenotypic mutants of certain Escherichia coli strains [ 5 ]. This phenomenon involving a tight contact between the donor cell and the recipient cell was called conjugation. In further experimental investigations conjugation could be seen to depend on the presence in the donor cell of a so-called fertility factor F, a relatively small autonomous DNA molecule [ 6 ]. Occasionally, F integrates into the bacterial chromosome to produce Hfr derivatives. Both in the autonomous and in the integrated state, F can give rise to pairs with F − recipient bacteria. This allows a copy of the replicating F to enter into the partner cell. From its integrated Hfr state, the F transfer normally also transfers neighboring parts of the donor chromosome. This kind of experiments led to the conclusion that the E. coli genome is one large circular DNA molecule. Occasionally, F undergoes unprecise excision from the Hfr chromosome. It thereby becomes autonomous again and carries in addition to its own genes some genes from its bacterial host. Such hybrid derivatives are called F’ and they are actually vectors for bacterial genes which can become transferred into recipient bacteria upon conjugation (see [ 6 , 7 ]).
F’-like conjugative plasmids have soon become known to act sometimes in the horizontal transfer of antibiotic resistance determinants. This can seriously contribute to the spreading of these determinants to pathogenic bacteria, in particular in the presence of antibiotics exerting a selective pressure.
2.3. Bacteriophage-Mediated Transduction
Transduction was first seen in studies on gene exchange between Salmonella bacteria [ 8 ]. In later investigations it became clear that several different kinds of bacterial viruses can occasionally also serve as natural vectors for genes of their host bacteria (see [ 7 ]). Two different strategies were identified to contribute to this horizontal transfer of genes between different host bacteria. In generalized transduction, some of the propagating progeny viral particles contain a segment from the host chromosome rather than a reproduced viral genome. This is, for example, seen for the Salmonella phage P22 and for the E. coli phage P1. In contrast, in specialized transduction, the transducing viral particle contains a hybrid molecule with a part of the phage genes and some bacterial genes. In the late 1950’s I had the chance to identify for the first time such a hybrid genome in λgal derivatives of phage λ. This hybrid DNA molecule was still able to undergo autonomous replication, but instead of some of its viral coat genes, it carried genetic determinants for the fermentation of galactose picked up in the host chromosome [ 9 ]. Its full reproduction depended therefore on the presence of an intact “helper” phage genome.
3. Bacterial Restriction/Modification Systems Limit Horizontal Gene Transfer
Upon genetic experimentation using several bacterial strains and their phages, a phenomenon called host-controlled modification was encountered by a few independent scientists. In the 1960’s we succeeded to unravel its molecular mechanisms [ 10 ]. In brief, many strains of bacteria possess one or even more than one genetic set-ups to identify invading foreign DNA as foreign and to start its destruction by endonucleolytic cleavage (restriction enzymes). If the invader is a bacteriophage, its DNA can escape restriction with a quite low probability. The viral genome becomes thereby insensitive to restriction by site-specific methylation (modification). This is a kind of epigenetic alteration that does not affect the genetic information carried in the phage genome. Reproduced modified phage then grows well without restriction in its new host, but often not any longer in its previous host. Restriction acts upon phage infection as well as upon transformation and conjugation between two bacterial strains carrying different restriction/modification capacities. We conclude that bacterial restriction/modification systems drastically limit successful horizontal gene transfer in the world of bacteria. This contributes to the genetic stability, but it still allows occasional genetic variation by horizontal gene transfer to occur as a contribution to biological evolution.
4. Other Natural Barriers against Functionally Relevant Horizontal Gene Transfer
In this context, it might be relevant to briefly mention here that in the world of bacteria and their viruses, several other barriers are known to limit the rates of uptake of foreign genetic information. Among these are a lack of bacterial surface compatibility for conjugational pair formation or for the uptake of DNA molecules from the medium, bacteriophage host range specificities, a requirement for functional harmony between the invading genetic information with the recipient’s genome, and a rather unexpected microbial immunity, called CRISPR-Cas, acquired upon a previous infection [ 11 ].
5. In Vitro Recombinant DNA Molecules and Their Use in Fundamental and in Applied Genetic Research
In view of the large size of DNA molecules carrying the genomic information of any organism, researchers reflected on possibilities to sort out particular genes and to multiply them in view of their structural and functional studies. Viral DNA molecules and F conjugative plasmids were envisaged to serve as autonomously replicating gene vectors, into which a relatively short DNA fragment could be spliced. Such hybrids might then serve for studies of both structural and functional properties of the inserted DNA fragment. This possibility became realistic when bacterial restriction enzymes became available around 1970 [ 12 ].
Scientists involved in this work discussed measures to be taken in order to prevent undesirable effects of recombinant DNA molecules with yet unknown genetic information. Such conjectural effects might affect the health of the investigator, on the one hand, and they might also render possible an undesirable spreading of the inserted genetic information to other organisms after an accidental or a deliberate release into the environment, on the other hand [ 13 ]. The answer to the latter concern required a better understanding of the laws of nature guiding spontaneously occurring genetic variation, the driving force of biological evolution.
6. Molecular Mechanisms and Natural Strategies of Genetic Variation
Both, already available data on spontaneous genetic variation and novel experimental results, in particular from microbial genetics, revealed a multitude of specific molecular mechanisms to contribute to the overall spontaneous genetic variation. We have classified these identified molecular mechanisms of genetic variation into three natural strategies of genetic variation [ 14 ]. These are: (a) Local alterations of nucleotide sequences, such as a nucleotide substitution, the insertion or the deletion of one or a few adjacent nucleotides. Such spontaneously occurring variations are often linked with DNA replication; (b) A second strategy of nature to produce genetic variations resides in a duplication, deletion, inversion or translocation of a DNA segment carried in the genome; (c) The third strategy of genetic variation consists in the acquisition by horizontal gene transfer of a segment of foreign DNA. Transformation, conjugation and viral infection can contribute to these effects. It is clear that the maintenance of any novel genetic variant is depending on Darwinian natural selection.
These natural laws of genetic variation are solidly shown to apply to microorganisms. Fewer experimental data are at this time available for higher organisms, but reports on cross-species gene transfer [ 15 ], as well as recent DNA sequence comparisons, speak clearly in favor of a general validity of the relevant natural laws of genetic variation for all living organisms.
6.1. Conceptual Implications of the Observed Laws of Genetic Variations
Experimental observations show that in spontaneously occurring genetic variation specific gene products are often involved, on the one hand, and/or non-genetic properties of the non-living world, on the other hand. Some of the involved gene products act as variation generators, others as modulators of the rates of genetic variation, which provides to the living organisms a relatively high genetic stability required for maintenance of life [ 14 ]. Many of the gene products required for a relatively slow but steady biological evolution are not essential for individual lives from one generation to the next. We therefore label their genetic information as evolution genes. As a philosophical consequence we note a dual nature of the genome. Many genes serve the individual life, while the evolution genes principally serve for the biological evolution of the population, a requirement for adaptation to changing habitats upon the terrestrial evolution of our planet [ 16 ].
In this self-organized biological evolution nature uses, besides the activities of evolution genes, a number of already mentioned non-genetic elements such as structural flexibilities (isomeric forms) and limited chemical stability of biological molecules, random encounter and environmental mutagens (chemicals and radiations). Random encounter is of particular relevance for horizontal gene transfer.
6.2. Implications of Horizontal Gene Transfer
The natural strategy to occasionally accept foreign genetic information by horizontal gene transfer links all living organisms into a global system. Whereas Darwin had drawn the evolutionary tree linking different organisms at the bottom of the tree, we can now, in addition, interlink different branches of the tree with horizontal connectors in order to allow for horizontal transfer of individual genes [ 17 ]. Being aware that horizontal gene transfer will also contribute to future events of genetic variation, we can conclude that the preservation of the encountered high biodiversity with a rich diversity of genetic information is essential and a guarantee for a harmonious long-term biological evolution on our planet. Note that a common language, i.e. , the universality of the genetic code, plays thereby an essential role [ 15 , 18 ].
6.3. Cohabitation Favors Horizontal Gene Transfer
In recent years it became obvious that all kinds of higher organisms, plants, animals and humans carry in and on their bodies a high number of microorganisms. These are only rarely pathogenic. They rather profit from a stable symbiosis. The higher organisms offer to bacteria good habitats, and bacteria contribute in a number of ways (food digestion, cleaning up of the skin, etc. ) to a healthy life of their hosts. This cohabitation is called microbiome and it can be maintained for long periods of time, whereby the microorganisms reside sometimes outside and in other cases inside of the cells of their host organism. Any such cohabitation must favor an occasional gene transfer between the involved organisms [ 18 ]. Such gene transfer can occur in both directions.
6.4. Evolutionary Inventiveness of Nature’s Self-Organization
Our present scientific insights into the evolution of life and of appropriate habitats reveal a remarkable degree of invention concerning different specific molecular processes that contribute to the generation of a rich diversity of forms of life living in a large number of different habitats. It becomes more and more known that many specific molecular mechanisms can contribute to a slow but steady evolutionary progress of living organisms. We have become aware that mechanistic insights into specific steps of genetic variation obtained in the work with one kind of bacteria cannot be generalized for all kinds of bacteria. On the other hand, it seems to us that the three defined natural strategies of genetic variation (local sequence change, intragenomic rearrangement of DNA segments, horizontal gene transfer) can best contribute to conceptually understand the self-organized natural process of the slow but steady evolution of life to a rich biodiversity within a global system of interdependencies. This does not only concern the genomic information, but also compositional differences of appropriate habitats, including the availability of healthy nutrition.
6.5. Time-Scale of Evolutionary Processes
In our daily life we are used to observe and to understand relatively fast processes. However, both the evolution of life and cosmic evolution are extremely slow, long-term processes which we have difficulty to perceive with our sensory organs. This may be a possible reason why a number of people (including some life scientists) still remain anchored in a fundamentalistic world-view claiming that there is no evolution. From an updated scientific view-point, we understand that a fast evolution of life could be detrimental. We assume that in the long past of biological evolution, the evolution gene activities of variation generators and of modulators of the rates of genetic variation have become fine-tuned to work as they do today. Note that spontaneous genetic variation is contingent so that by far not all genetic variants show improved functions [ 19 ]. Therefore, a rapid evolutionary progress would rather lead to an eradication of forms of life than to a steady contribution to enrich the treasure of biodiversity.
6.6. The Natural Strategies of Genetic Variation Contribute to Biological Evolution with Different Qualities
Let us start with horizontal gene transfer, i.e. , the acquisition of genetic information that had been developed in other kinds of living organisms. By chance, a gene acquisition can provide to the receiving organism a welcome novel function that provides a selective advantage. An example is the acquisition of genetic information for antibiotic resistance by pathogenic bacteria. We see here a sharing in the evolutionary success of other organisms. Of course, horizontal gene transfer can, in other cases, also lead to a selective disadvantage by negative impacts on the functional harmony of the receiving organism.
The quality of local mutagenesis resides mainly in a possible stepwise improvement of an already existing biological function. Again, such mutations are contingent and not precisely targeted as a response to identified needs.
Intragenomic DNA rearrangements offer possibilities to fuse functional segments of DNA sequences; this can occasionally result in novel activities. The fusion between two different functional domains may, by a rare chance, lead to a novel, welcome function. Or the fusion of a functional gene with an alternative control element for gene expression can influence the expression of the concerned gene.
We have to be aware that in all these events, functional improvements can be a relatively rare consequence, while a selective disadvantage and in the extreme case lethality are more likely to result. The self-organizing biological evolution requires thereby a sacrifice from one individual in a large population undergoing a slow but steady evolution. Let us mention in this context that religious persons may encounter conceptual difficulties to accept that genetic adaptation to novel life conditions occurs with some contingency.
7. The Role of Horizontal Gene Transfer in Synthetic Biology
As we have already outlined above, in the recombinant DNA technique selected DNA sequences are sorted out and spliced into other DNA molecules such as natural gene vectors. This and other procedures, such as site-directed mutagenesis, have become important contributions to the so-called synthetic biology that can serve for useful applications of fundamental scientific knowledge on biological functions. Insights into principles of natural strategies of genetic variation as outlined in Section 6 can thereby help to prevent conjectural risks of experimentation and of its products [ 20 ]. A general advice of relevance is the recommendation that natural laws of genetic variation as discussed in Section 6 , should be respected in the in vitro construction of genomic segments. With regard to the formation of recombinant DNA molecules, this precautionary principle can validly contribute to the biosafety of the envisaged product of biosynthesis. Interesting examples of in vitro transfer of useful genetic information into another living organism allowing for a domesticated production and harvest of the relevant gene product were reported recently [ 21 ]. We can predict that future investigations will continue to offer welcome availabilities of identified genes and of their products which can serve for beneficial use to the service of humankind and of its environment. It is likely that horizontal gene transfer will thereby continue to play a major role.
Supplementary Files
Supplementary file 1.
Review Report (PDF, 137 KB)
Conflicts of Interest
The author declares no conflict of interest
Loading metrics
Open Access
Essays articulate a specific perspective on a topic of broad interest to scientists.
See all article types »
Diversity Takes Shape: Understanding the Mechanistic and Adaptive Basis of Bacterial Morphology
Contributed equally to this work with: David T. Kysela, Amelia M. Randich
Affiliation Department of Biology, Indiana University, Bloomington, Indiana, United States of America

* E-mail: [email protected]
- David T. Kysela,
- Amelia M. Randich,
- Paul D. Caccamo,
- Yves V. Brun
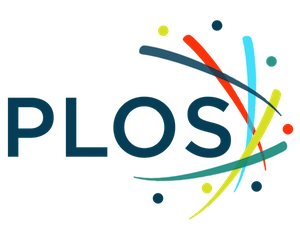
Published: October 3, 2016
- https://doi.org/10.1371/journal.pbio.1002565
- Reader Comments
The modern age of metagenomics has delivered unprecedented volumes of data describing the genetic and metabolic diversity of bacterial communities, but it has failed to provide information about coincident cellular morphologies. Much like metabolic and biosynthetic capabilities, morphology comprises a critical component of bacterial fitness, molded by natural selection into the many elaborate shapes observed across the bacterial domain. In this essay, we discuss the diversity of bacterial morphology and its implications for understanding both the mechanistic and the adaptive basis of morphogenesis. We consider how best to leverage genomic data and recent experimental developments in order to advance our understanding of bacterial shape and its functional importance.
Citation: Kysela DT, Randich AM, Caccamo PD, Brun YV (2016) Diversity Takes Shape: Understanding the Mechanistic and Adaptive Basis of Bacterial Morphology. PLoS Biol 14(10): e1002565. https://doi.org/10.1371/journal.pbio.1002565
Copyright: © 2016 Kysela et al. This is an open access article distributed under the terms of the Creative Commons Attribution License , which permits unrestricted use, distribution, and reproduction in any medium, provided the original author and source are credited.
Funding: Research reported in this publication was supported by the National Institute of General Medical Sciences of the National Institutes of Health under award number R01GM51986 to YVB and by National Research Service Award F32GM112362 to AMR. The content is solely the responsibility of the authors and does not necessarily represent the official views of the National Institutes of Health. The funders had no role in study design, data collection and analysis, decision to publish, or preparation of the manuscript.
Competing interests: The authors have declared that no competing interests exist.
Abbreviations: FDAA, fluorescent D-amino acid; PG, peptidoglycan
Introduction
Imagine a bacterium. Did you imagine asymmetric, multicellular filaments of curved bacteria, such as those belonging to the genus Simonsiella ( Fig 1.21 )? These bacteria glide slowly on the surface of your palate using the concave side of their curved cells and divide parallel to their long axis [ 1 ]. Or perhaps instead you imagined a photosynthetic, ovoid bacterium like Rhodomicrobium vannielii , which grows extensions of the inner membrane, cell wall, and outer membrane ( Fig 1.19 )? From its elongating (and sometimes branching) extensions, this bacterium can bud one of three types of cells: appendaged cells like itself; swimming cells; or angular, heat-resistant exospores [ 2 ]. The appendaged daughter cells, remaining attached to the mother cell, grow their own extensions and progeny to create giant networks of connected cells in the mud.
- PPT PowerPoint slide
- PNG larger image
- TIFF original image
Bacterial phylogeny derived from genome sequence data for selected species, with an emphasis on morphologically and phylogenetically diverse taxa. Sequence data gathered from the Joint Genome Institute [ 3 ] and the National Center for Biotechnology Information [ 4 ] were searched for reference genes and aligned using Phylosift [ 5 ]. FastTree [ 6 ] generated an approximate maximum likelihood tree from the resulting concatenated alignment. The final tree was formatted using iTol [ 7 ]. Black dots denote ancestral nodes of selected major taxa: DT , Deinococcus-Thermus; Ac , Actinobacteria; Cf , Chloroflexi; Cn , Cyanobacteria; Fi , Firmicutes (inclusive of Mollicutes); Sp , Spirochetes; PVC , Planctomycetes, Verrucomicrobia, Chlamydiae; Cb , Chlorobi; Bd , Bacteroidetes; α , β , γ , δ , ε , Proteobacteria subdivisions. 1. Bifidobacterium longum . 2. Streptomyces coelicolor (mycelial [multicellular] filament with hyphae and spores). 3. Corynebacterium diphtheria e (two cells, dumbbell and club shapes). 4. Herpetosiphon aurantiacus (filament of multiple cylindrical cells). 5. Calothrix (filament of multiple disk-shaped cells). 6. Mycoplasma genitalium . 7. S piroplasma culicicola . 8. Lactococcus lactis (predivisional cell). 9. Borrelia burgdorferi . 10. Gimesia maris (previously Planctomyces maris , predivisional cell with proteinaceous stalk). 11. Prosthecochloris aestuarii . 12. Pelodictyon phaeoclathratiforme (filament of multiple trapezoidal cells). 13. Spirosoma linguale . 14. Muricauda ruestringensis (appendage includes nonreproductive bulb). 15. Desulfovibrio vulgaris (two cells, helical and curved shapes). 16. Helicobacter pylori . 17. Caulobacter crescentus (predivisional cell). 18. Hyphomonas neptunium (predivisional cell) . 19. Rhodomicrobium vannielii (filament of multiple ovoid cells, one is predivisional) . 20. Prosthecomicrobium hirschii . 21. Simonsiella muelleri (filament of multiple curved cells). 22. Nevskia ramosa (two cells with bifurcating slime stalk) . 23. Beggiatoa leptomitiformis (filament of multiple, giant cylindrical cells). 24. Thiomargarita nelsonii (single, giant cell). 25. Escherichia coli . 26. Mariprofundus ferrooxydans (single cell with metal-encrusted stalk). Bacterial schematics are not to scale. Species names are colored according to morphology as indicated in the key. Colored dots are appended to indicate species with multiple morphologies. Names of species depicted in schematics are emphasized in large, bold font.
https://doi.org/10.1371/journal.pbio.1002565.g001
Or did you imagine a rod; in particular, one that elongates to double its length and then divides in two?
Perusing the once-definitive guide to bacterial identification, Bergey’s Manual of Determinative Bacteriology , one easily finds shapes much more interesting than rods and cocci [ 8 ]. Even the language used to describe the morphologies of various species in the text quickly illustrates the veritable bacterial zoo found on earth: In addition to the familiar coccoid, rod-shaped, or spirillar types, there are also dendroid, coryneform, cylindrical, bulbiform, fusiform, and vibrioid types. There are uniseriate or multiseriate filaments of cells that are flexible or rigid, flat or round, unbound or bound in hyaline or slime sheaths. Single cells are described as star-shaped, disk-shaped, hourglass-shaped, lemon-shaped, pear-shaped, crescent-shaped, or flask-shaped. Rods can be pleomorphic, straight, curved, or bent, with blunt, pointed, rounded, or tapered ends. Some cells grow appendages such as prosthecae, stalks, or spikes. The representative schematics in Fig 1 offer a glimpse of some of this diversity but hardly do justice to the variation of size and shape across the bacterial domain. Bergey’s served as a guide for identifying species phenotypically for a century, underscoring how reliably each species reproduces its signature morphology.
A curious reader of Bergey’s may find it perturbing that the more unusually shaped bacteria comprise a minority of the book, and most of the micrographs and notes on them date from before 1980. If morphological diversity is so pervasive, why do rods and cocci dominate the manual? And why is the information so old? These deficiencies not only reveal the historical focus of the field of microbiology on pathogenic bacteria, which tend to be rods and cocci, but also the shift in interest of the field to model organisms on the advent of molecular biology. During the 1970s, significant progress was made in gaining genetic control over Escherichia coli , thereby establishing it as the model bacterium [ 9 , 10 ]. Since that era, model bacteria such as E . coli and Bacillus subtilis have dominated research because of their genetic tractability and culturing ease. Many of the more strangely shaped bacteria proved unculturable, or their original strains were lost. In effect, Bergey’s serves as some sort of time capsule from which it is clear that a great diversity of bacterial morphologies exists. Sadly, this diversity is still likely to be highly undersampled, as the high-throughput metagenomic approaches that are quickly filling out the bacterial domain do not capture morphological data. A more complete visual survey of the bacterial domain would reveal more morphologies, the number of species with atypical morphotypes might rival those of the known rods and cocci, and those “typical” rods and cocci would exhibit a great deal more morphological variability than currently projected by the field. How are these diverse morphologies related evolutionarily and mechanistically, and what are their functions?
Morphology and Bacterial Evolution
Phylogenetic trees based on molecular sequence data have transformed how we understand bacterial evolutionary relationships [ 11 ]. Such phylogenies have proven that the historical taxonomic approach used to classify bacteria based on phenotypes such as morphology often grouped bacteria unrelated by descent. For example, the Betaproteobacteria Rhodocyclus tenuis and Rubrivivax gelatinosus were misclassified as members of the genus Rhodospirillum , and therefore as Alphaproteobacteria, partially on the basis of their helical shape [ 12 ]. Clearly, even careful and expert interpretation of phenotypes alone can lead to misinterpretations of relatedness between species. In another case, appendages called prosthecae, which are thin extensions of the entire cell envelope, were considered a unifying characteristic of the genus Prosthecomicrobium . However, 16S phylogenetic analysis led to the split of the single genus into three separate genera that were each more closely related to a nonprosthecate genus than to one another [ 13 ]. Thus the prosthecate morphology most likely was shared by a common ancestor to this group and was lost in some lineages. Together, these examples suggest that (1) similar-looking morphologies can and do evolve independently in unrelated genera and that (2) the histories of certain morphologies among related genera can be complex.
Mapping our current, yet limited, knowledge of morphological phenotypes onto robust phylogenies allows us to make inferences about how bacterial shapes evolved. This approach has seen extensive application for inferring the evolutionary history of traits in eukaryotes [ 14 ], and it was similarly applied to exploring transitions from rod to coccus shapes in the bacterial domain [ 15 ]. Using updated phylogenetic tools, we have constructed a phylogeny that focuses on diverse morphologies beyond rods and cocci ( Fig 1 ). Some morphologies, such as helical cells (green) or multicellular filamentous types (red), appear repeatedly throughout the bacterial domain. This scattering amongst distant clades indicates that these morphologies have several, independent evolutionary origins. In some cases, the same morphology appears to cluster in a specific region of the phylogenetic tree, such as branching in the Actinobacteria (purple) or appendages in the Caulobacterales (blue). This clustering indicates persistence of a morphology inherited from a shared ancestor. These relationships between phylogeny and morphology generate many questions important to evolutionary biology and bacteriology. When the same shape arises independently, different lineages with distinct constraints somehow arrive at a common morphology: Are these shapes generated via similar molecular strategies? Are they influenced by similarities in the environment or bacterial lifestyle? In cases of shared ancestry, we can address the persistence of a specific morphology: Is the shape retained through continued selective pressure? How and why does morphological variation among family members arise? We are only beginning to answer these questions. However, our developing perspective on bacterial diversity and the ability to map morphologies onto the ever-growing phylogenetic tree of the bacterial domain will allow us to better address them.
The reason that bacteria have certain shapes remains unclear; we can only surmise that certain shapes have adaptive value or have been produced by other selective forces since they are under genetic control and are maintained from generation to generation. Many theories offer rationales for the adaptive value of specific morphologies [ 16 , 17 ]. Young’s [ 16 ] excellent review explores the relationship of selective forces and bacterial morphology in detail that goes well beyond the scope of this essay. In general, morphological traits can be attributed to adaptation to selective forces such as nutrient limitation, reproduction, attachment, dispersal, and evasion of predation or host detection. In the simplest formulations, helical and curved cells appear to be optimized for motility, especially in viscous solutions [ 18 – 21 ], large cells (and very small cells) for evading ingestion (or capture) by protists [ 22 , 23 ], and branching and net-forming cells for buoyancy and soil retention [ 24 , 25 ].
We can easily speculate on the adaptive value of the morphologies presented in Fig 1 . Take for example the extremely long (100–1,200 μm) multicellular filaments of Herpetosiphon aurantiacus ( Fig 1.4 ), which are capable of rapid gliding motility and remarkable flexibility [ 26 , 27 ]. It is possible that the extraordinary lengths of these filaments allow this bacterium to evade phagocytosis by protists or boost its gliding motility on surfaces. Perhaps the flexibility of the multicellular filaments allows entwinement with various substrates in aqueous or soil environments to enhance adherence and retention. Even more exciting, these traits may play an important role in its facultative predation of other bacteria, in which its looped filaments trap and “bulldoze” other bacteria, lysing them with secreted hydrolases [ 28 ]. Or, consider the case of Pelodictyon phaeoclathratiforme ( Fig 1.12 ), a filamentous green sulfur bacterium that forms three-dimensional nets of trapezoidal cells via branching and possibly ternary division [ 29 , 30 ]. Arguably, these nets slow sedimentation and help to keep colonies of this bacterium at the correct level in the water column, where they are optimally situated between opposing gradients of light and sulfide [ 31 ]. Or, take for example the extreme size of members of the genus Thiomargarita ( Fig 1.24 ), a coccus that averages 0.1–0.3 mm in diameter [ 32 – 34 ]. This bacterium breaks the usual limitations of diffusion on size by harboring a nitrate-rich, liquid-filled vacuole that takes up 80%–98% of the cell volume and leaves only a 0.5–2 μm layer of cytoplasm [ 32 ]. The large size of Thiomargarita has been posited as an adaptation for a nonmotile lifestyle in which periodic resuspension of the large, buoyant cells puts them in contact with the oxygenated water column [ 33 , 34 ]. The vacuole, as well as a host of cytoplasmic sulfur inclusions, may also serve as a nutrient reservoir, which enables the bacterium to maintain metabolism during nutrient limitation between resuspension events.
Adaptive explanations for bacterial morphology provide a convenient narrative, but we must also account for alternatives. Consider the proposed roles of Herpetosiphon ’s multicellular filamentation: gliding motility, predation defense, and its own predatory behavior. The shape may serve an adaptive role in just one of these functions or some combination thereof. However, all these guesses may be off the mark. Perhaps multicellular filamentation instead provides a different function, such as clonal cohesion in order to reinforce cooperative behavior like nutrient scavenging [ 35 ]. Or, the observed morphology may arise merely by chance rather than adaptation [ 36 ]. Alternatively, multicellular filamentation may arise as a byproduct of selection for a different phenotype [ 37 ], such as enhanced surface attachment that also happens to increase attachment between cells. All too often, adaptive explanations for morphology comprise quaint, just-so stories, residing unchallenged at the end of an article’s discussion section. Validating adaptive hypotheses for morphology requires the same scrutiny applied to other scientific problems: generating and testing a clear, falsifiable hypothesis. Only with clear, empirically tested hypotheses regarding the selective function of shape can we begin to paint a clearer picture regarding the environmental pressures that have shaped the historical evolution of the diverse array of observed bacterial morphologies.
Mechanisms of Morphogenesis
Although it can be very difficult to determine why bacteria have certain morphologies, many inroads have been made into determining how they obtain them. The vast majority of bacteria synthesize a peptidoglycan (PG) cell wall that provides structural integrity to the cell. The growth, maintenance, and modification of the cell wall play a key role in defining the shape of the cell [ 38 ]. Much of what we know regarding bacterial shape determination at the molecular level in bacteria comes from the study of a few model organisms with relatively simple morphologies, including the following: the sphere or ovoid shape of Streptococcus pneumoniae or Staphylococcus aureus ; the rod shape of E . coli , B . subtilis , or Agrobacterium tumefaciens ; and the spiral shape of Helicobacter pylori . These model systems have proven experimentally advantageous in understanding basic and common principles involved in different modes of bacterial cell growth. A conserved set of proteins participates in PG synthesis and remodeling [ 39 , 40 ], thus the details of cell wall synthesis gathered from select model systems provide a solid touchstone for exploring even divergent morphologies.
Bacteria must remodel the cell wall in order to grow and divide, and this activity underlies their shape-generating capacity. Because PG synthesis is constrained in space, all cell wall growth and remodeling can be described as “zonal” growth at the molecular level [ 41 ]. Zonal growth at specific points during the cell cycle gives rise to specific patterns of PG synthesis, or growth modes. A number of growth modes have been shown to generate the rod shape. The best-studied growth mode, exemplified by B . subtilis , is lateral elongation, in which PG synthesis is evenly dispersed along the length of the cell. E . coli and Caulobacter crescentus utilize lateral elongation as well as an additional growth mode called preseptal or medial elongation, in which PG synthesis occurs at the midcell. Alternatively, some bacteria, such as A . tumefaciens , employ polar elongation at one or both poles [ 42 ]. Despite differences in the specific details of each system at the molecular level, all these growth modes can be viewed as a modular, spatiotemporal utilization of zonal growth to create the rod shape. But how does zonal PG synthesis generate diverse shapes beyond simple rods and cocci? Conceptually, simply repositioning zonal growth to specific locations could generate a diverse range of morphologies derived from the basic rod shape. Take as an example the Caulobacter prostheca, which results from zonal growth restricted to the cell pole ( Fig 2A ). In the closely related genus, Asticcacaulis , distinct prosthecate morphologies arise from repositioning the growth zone(s) at subpolar and bilateral sites ( Fig 2B and 2C ) [ 43 , 44 ]. In Actinobacteria, branching results from repositioning the polar growth PG machinery to create new growing poles ( Fig 2D ) [ 45 ]. Thus, simple variations on the theme of zonal PG growth can give rise to diverse morphologies.
(A–C) In prosthecate Alphaproteobacteria, simply repositioning zonal PG synthesis machinery (shown in green) can generate polar ( Caulobacter crescentus , A ), subpolar ( Asticcacaulis excentricus , B ), or bilateral ( Asticcacaulis biprosthecum , C ) prosthecate phenotypes. (D) Similar repositioning of the PG growth zone yields a branching phenotype in Streptomyces coelicolor , which grows from the cell poles. (E) The intermediate filament-like protein crescentin (shown in cyan) of C . crescentus constrains PG synthesis to generate cell wall curvature. (F) When cells filament, this constraint results in long helical shapes. (G) The periplasmic flagella of Borrelia burgdorferi directly deform the cell body into a planar wave shape. Note that the scale of the periplasmic space, relative to the cell membranes, has been modified to highlight this arrangement. For simplicity, details of the periplasmic compartment are shown only for panel G .
https://doi.org/10.1371/journal.pbio.1002565.g002
While similar growth mechanisms may yield diverse morphologies among close relatives, can shared mechanisms also explain similar morphologies in phylogenetically distant bacteria? An interesting comparison can be made between the alphaproteobacterium C . crescentus and the spirochete Borrelia burgdorferi , which are only very distantly related. C . crescentus exhibits a vibrioid (curved) morphology, which gives rise to helical shapes when the cells filament ( Fig 2E and 2F ) [ 46 ]. This morphology requires the species-specific molecular scaffold crescentin, which imposes a structural constraint along the inner curve of the cell to guide PG synthesis [ 46 , 47 ]. In contrast, cell curvature in the spirochete B . burgdorferi follows different rules, relying on a structural role of periplasmic flagella. In this case, what may appear to be a helical morphology instead comprises a “flat wave” shape created by the constraints of the periplasmic flagella ( Fig 2G ). Rather than inducing curvature via differential PG synthesis, the periplasmic flagella are posited to directly deform the cell body to generate curvature [ 48 ]. Despite their mechanistic differences, both C . crescentus and B . burgdorferi generate curvature according to a common structural theme: scaffold-mediated delineation of an inner curvature. It will be interesting to see whether other examples of evolutionarily distinct curvature and helicity depend on similar themes. Do all curved cells require a scaffold? Do such scaffolds share particular physical or biochemical properties? Indeed, cytoplasmic scaffolding proteins (in addition to PG-modifying enzymes [ 49 , 50 ]) participate in helical morphogenesis in H . pylori [ 51 , 52 ]. Like crescentin, these proteins self-oligomerize and contain coiled-coil motifs known to participate in protein–protein interactions. Although detailed experimentation is required in order to identify the components used to achieve morphogenesis in particular species, unraveling these mechanistic details can help to identify general morphogenetic strategies that may reveal fundamental constraints on how particular shapes evolve. These general trends can guide our investigations of morphogenetic mechanisms in other bacteria and enhance our understanding of how bacterial shape evolves.
A Path Forward
Although microbiologists have long appreciated the impressive morphological diversity among bacteria, we still have much to learn. Modern technologies provide access to unprecedented quantities of data, particularly microbial genome sequences. However, harnessing these data to address the “how” and “why” of morphological evolution presents a challenge.
We must first consider where to direct our efforts in order to learn about morphology. A clear picture of morphological evolution requires a representative sample of phylogenetic diversity thorough enough to identify important morphological transitions. This picture becomes muddied through oversampling of pathogens and other species of applied significance [ 53 ]. Repeatedly studying similar, closely related bacteria effectively resamples the same ancestral events [ 54 ]. Phylogenetically informed sampling helps to resolve this problem by identifying unique morphological transitions in the bacterial tree. More fundamentally, overemphasis on particular taxa limits our perspective on representative morphological variation across the bacterial domain. Recent genome sequencing efforts emphasize more phylogenetically comprehensive sampling, particularly the Genomic Encyclopedia of Bacteria and Archaea [ 55 ]. This approach has helped to span various independent morphological transitions among available genome sequences ( Fig 1 ). Importantly, much of the bacterial domain remains uncharacterized [ 56 ], and numerous morphological variants likely await discovery. High-throughput culturing [ 57 ] generates relatively unbiased bacterial libraries from an environment of interest. High-content imaging [ 58 ] and automated image analysis provides an unbiased quantification of morphological parameters [ 59 – 61 ] to identify bacteria of morphological interest. Even among the very large fraction of bacteria that remain unculturable, the advent of single-cell genomics opens the door to sequencing genomes of morphologically relevant species [ 62 ]. This approach has already successfully paired high-resolution microscopy with whole-genome sequences of noncultured bacteria [ 63 ]. These strategies will help to provide a more complete picture of morphological diversity and characterize key morphological transitions that best inform our understanding of how bacterial shape evolves.
Our laboratory used such a phylogenetically informed approach in order to understand the molecular basis of the prosthecate morphology in the model organism Caulobacter and closely related genera. The phylogeny of Caulobacter , Brevundimonas , and Asticcacaulis ( Fig 3 ) indicates that an ancestral polar prostheca has been repositioned (first subpolar and then lateral in Asticcacaulis ), increased in number ( Asticcacaulis biprosthecum ), and lost outright ( C . segnis , Brevundimonas diminuta ). We recently examined the morphological transition from a polar prostheca to subpolar or lateral prosthecae. Specifically, we determined a morphogenetic function for the protein SpmX, previously shown to participate in developmental regulation in C . crescentus [ 64 ]. In Asticcacaullis , SpmX has been co-opted to position and coordinate prostheca PG synthesis [ 43 ]. An expanded region within this protein appears to be responsible for the difference in localization patterns between A . excentricus (subpolar) and A . biprosthecum (bilateral). This particular story of morphogenesis mechanism and evolution emerged from a combination of two methods: comparative study of various Asticcacaulis and Caulobacter species and direct genetic manipulation informed by the well-developed Caulobacter experimental model. In this case, progress was possible because we utilized a model organism and its close relatives. However, many morphologically interesting species are poorly studied, with little in the way of prior research or available genetic tools. Is the study of bacterial morphology then stuck relying on a limited selection of existing, extensively developed experimental models and their close relatives? Thankfully, things are not so grim; comparative molecular genetics, automated image analysis, and advanced labeling tools all offer interesting solutions for studying morphology in underrepresented systems.
(A) Phylogeny of the order Caulobacterales generated as described in Fig 1 . Schematics and corresponding colors indicate inferred ancestral morphologies and their subsequent inheritance. Black branches indicate rod-shape, nonappendaged morphology, including several apparent prostheca loss events. Scale bar indicates 0.1 amino acid substitutions per site. (B) Transmission electron micrographs of members of the Caulobacterales, highlighting disparate prosthecate morphologies. For each morphology, a brief description and the name of one representative species is provided, followed by the image source in parentheses. 1. Bilateral prosthecae, Asticcacaulis biprosthecum (Chao Jiang, Stanford University). 2. Subpolar prostheca, Asticcacaulis excentricus (Chao Jiang, Stanford University). 3. Polar prostheca, Caulobacter crescentus (Paul Caccamo, Indiana University). 4. Polar prostheca, Maricaulis maris (Patrick Viollier, University of Geneva). 5. Short polar prostheca, Brevundimonas subvibriodes (Brynn Heckel, Indiana University); note other members of this genus display a much longer prostheca. 6. Polar prostheca through which budding reproduction occurs, Hirschia baltica (Paul Caccamo, Indiana University). Magnification varies between micrographs. All images are reproduced with permission.
https://doi.org/10.1371/journal.pbio.1002565.g003
Genome sequence data can provide significant insight into the evolution of morphology. From sequence data, comparative molecular genetics can identify patterns of gene content, selection on individual genes (including individual residues), and functionally connected suites of genes [ 65 , 66 ]. Applying this approach to existing genome sequence data may reveal the selective targets associated with specific morphologies. Indeed, this comparative genomic strategy identified specific proteins associated with the transition from rods to cocci in pathogenic bacteria [ 67 ]. Of course, genomic data cannot substitute for testing morphological mechanisms by direct experimentation. Nonetheless, comparative genomics can help to identify suitable targets for empirical study.
Modern tools also facilitate direct morphological experimentation, even for species without previous experimental development. In particular, recently described fluorescent D-amino acids (FDAAs) label sites of cell wall synthesis in diverse bacteria by leveraging the ubiquity of PG [ 68 , 69 ]. This labeling strategy enables precise, microscopic observation of the growth modes underlying interesting morphologies, even for bacteria that are poorly understood or experimentally intractable. Another valuable tool, high-throughput imaging, can identify morphological determinants even in the absence of advanced molecular genetic tools. Imaging mutant libraries can identify relevant protein targets by screening for specific phenotypes such as the following: (1) morphological variants in libraries generated by transposon or chemical mutagenesis and (2) proteins localized to morphological features in fluorescent transposon fusion libraries. Finally, automated analysis of cell images provides efficient and quantitative analysis of large mutant libraries [ 59 – 61 ]. These tools permit relatively detailed analysis of bacterial morphogenesis in model and nonmodel organisms alike.
We propose an experimental strategy for leveraging these tools to study novel morphogenetic mechanisms in order to determine both how patterned cell growth generates specific morphologies and the underlying molecular machinery. Pulse labeling with FDAAs reveals the cell wall growth pattern responsible for generating a particular bacterial shape [ 68 ]. Such underlying growth patterns are not always obvious from the final cell shape, even for relatively simple shapes like rods [ 42 ]. After growth patterns have been determined, the next goal is to identify genetic loci that participate in morphogenesis. Mutant libraries can be generated through transposon or chemical mutagenesis and then screened by high content imaging and automated image analysis for variants in shape and FDAA labeling patterns. Sequencing of the mutated site (transposon) or of the whole genome (chemical mutagenesis) can then identify affected loci. This broadly applicable experimental strategy can reveal both underlying growth patterns and mechanistic components responsible for generating particular morphotypes. Applying this strategy across diverse taxa and morphologies can identify common themes and points of departure in mechanisms of morphogenesis. By casting a wide net, such observations can guide further development of key model organisms for careful inquiry at the molecular level.
Even as we unravel the mechanistic details of bacterial morphogenesis, the ultimate explanation for the varied shapes observed in nature derives from the selective forces at play in the environment. Complex morphologies like spirals and appendages do not emerge and persist for millions of years by chance alone. However, as discussed earlier, determining the adaptive value of shape requires moving beyond telling plausible, “just so” stories. Fortunately, evolutionary biology already provides a theoretical and experimental framework for directly examining how morphology influences bacterial fitness.
Compelling evidence of the adaptive value of shape requires direct observation of selection acting on phenotype. The most direct evidence of adaptive value derives from head-to-head competition of different morphotypes and the emergence of a superior competitor via selection. A recent study employed pairwise competition of distinct E . coli cell size mutants, identifying fitness effects of cell size that depend on the particular growth environment [ 70 ]. Similarly, direct competition among morphotypes of H . pylori has proven that native cell curvature enhances stomach colonization when compared against mutants with reduced curvature [ 49 ], demonstrating that shape is a key fitness component for a pathogen. Future work should follow a similar path in establishing adaptive relevance of morphology, varying both morphotype and environment while measuring fitness through direct competition.
We envision a combined strategy of direct competition assays and laboratory evolution for testing hypothesized functional significance of particular bacterial morphologies. Based on a hypothesized adaptation to a specific environmental constraint, competition assays should identify a selective advantage of the adaptive morphotype over its mutant competitors, but only when the constraint is imposed. Let us return to the example of Pelodictyon , whose branched cell networks hypothetically enhance fitness by retarding sedimentation and maintaining correct cell position along light and sulfite gradients. According to this sedimentation hypothesis, wild-type cells should readily outcompete a non–net-forming mutant in an appropriately spatially structured laboratory environment. However, agitation to disrupt spatial environmental gradients should abolish the wild-type competitive advantage conferred by retarded sedimentation. Failure of the wild type to outcompete the mutant in the structured environment would suggest an alternate adaptive role of the branched cell network morphology. Additionally, we can use experimental evolution to observe natural selection of morphology directly in the laboratory. If selection for reduced sedimentation maintains the filamentous network morphology of Pelodictyon , selection of Pelodictyon in agitated (unstructured) environments should result in the eventual erosion of the morphology via genetic drift, whereas continued selection in a structured environment should preserve the native phenotype. Although we cannot reconstruct historical environmental pressures with certainty, experiments like these can provide empirical support for adaptive hypotheses. This proposed strategy for testing the functional basis of morphology elevates adaptive hypothesis beyond throwaway comments in the discussion and into their rightful position among the results.
Decades after an initial surge in environmental microbiology described a vast array of different bacterial shapes, we are left with an impressive body of knowledge describing what is out there, but relatively little in the way of how and why. While much remains unresolved regarding the details of bacterial morphogenesis, the current scientific landscape affords great opportunity for progress. By pairing abundant genome sequence data with modern tools for imaging cell growth, new experimental approaches are now available for morphologically diverse bacteria, even in cases in which little prior research exists. We hope this opportunity yields new insights and renewed interest in certain less familiar, morphologically varied bacteria that lie beyond rods and cocci.
Supporting Information
S1 fig. high-resolution version of fig 1 ..
Vector graphics format version of Fig 1 .
https://doi.org/10.1371/journal.pbio.1002565.s001
Acknowledgments
The authors thank Jim Staley for thoughtful discussion and members of the Brun laboratory for comments on the manuscript. The authors dedicate this essay to the memory of Arthur Koch (1926–2016), a pioneer in investigating the mechanisms of bacterial growth, morphogenesis, and evolution.
- View Article
- PubMed/NCBI
- Google Scholar
- 8. Bergey DH, Holt JG. Bergey's manual of determinative bacteriology. 9 ed. Baltimore: Williams &Wilkins; 1994.
- 26. Lee N, Reichenbach H. The Genus Herpetosiphon . In: Dworkin M, Falkow S, Rosenberg E, Schleifer K-H, Stackebrandt E, editors. The Prokaryotes: Volume 7: Proteobacteria: Delta, Epsilon Subclass. New York, NY: Springer New York; 2006. p. 854–77.
- 33. Schulz HN. The Genus Thiomargarita . In: Dworkin M, Falkow S, Rosenberg E, Schleifer K-H, Stackebrandt E, editors. The Prokaryotes: Volume 6: Proteobacteria: Gamma Subclass. New York, NY: Springer New York; 2006. p. 1156–63.
- 37. Wright S. Evolution and the genetics of populations; a treatise. Chicago: University of Chicago Press; 1968.
Fundamentals of Bacterial and Viral Genetics
Cite this chapter.
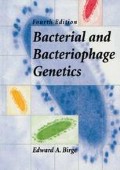
- Edward A. Birge 2
393 Accesses
1 Citations
Before beginning the formal study of the genetics of bacteria and their viruses, it is important to have a clear understanding of the fundamentals of microbial cell structure and of basic viral processes. This chapter briefly reviews cell anatomy as well as genetic terminology and processes with respect to bacteria, yeast, and viruses. More detailed coverage of major genetic processes is provided in later chapters. However, these summaries are essential for understanding some of the experiments presented in the next few chapters.
This is a preview of subscription content, log in via an institution to check access.
Access this chapter
- Available as PDF
- Read on any device
- Instant download
- Own it forever
Tax calculation will be finalised at checkout
Purchases are for personal use only
Institutional subscriptions
Unable to display preview. Download preview PDF.
Brock, T.D. (1990). The Emergence of Bacterial Genetics . Cold Spring Harbor, NY: Cold Spring Harbor Laboratory Press. (Historical review of the field.)
Google Scholar
Demerec, M., Adelberg, E.A., Clark, A.J., Hartman, P.E. (1966). A proposal for a uniform nomenclature in bacterial genetics. Genetics 54: 61–76.
PubMed CAS Google Scholar
Lederberg, J. (1987). Genetic recombination in bacteria: a discovery account. Annual Review of Genetics 21: 23–46.
Article PubMed CAS Google Scholar
Perry, J.J., Staley, J.T. (1997). Microbiology: Dynamics and Diversity . Fort Worth, TX: Saunders College Publishing. A general text suitable as a tool for review.
Schaechter, M. (1990). The bacterial equivalent of mitosis. In: Drlica, K., Riley, M. (eds.) The Bacterial Chromosome . Washington, DC: American Society for Microbiology, pp. 313–322. (A brief overview of recent experimental results.)
Thaler, D.S., Roth, J.R., Hirschbein, L. (1990). Imprinting as a mechanism for the control of gene expression. In: Drlica, K., Riley, M. (eds.) The Bacterial Chromosome . Washington, DC: American Society for Microbiology, pp. 445–456. (A discussion of the behavior of diploid bacterial cells.)
Wake, R.G., Errington, J.A.F. (1995). Chromosome partitioning in bacteria. Annual Review of Genetics 29: 41–67.
Specialized
Akamatsu, T., Sekiguchi, J. (1987). Genetic mapping by means of protoplast fusion in Bacillus subtilis. Molecular and General Genetics 208: 254–262.
Gordon, G.S., Sitnikov, D., Webb, C.D., Teleman, A., Straight, A., Losick, R., Murray, A.W., and Wright, A. (1997). Chromosome and low copy plasmid segregation in E. coli : Visual evidence for distinct mechanisms. Cell 90: 1113–1121.
Lévi-Meyrueis, C., Sanchez-Rivas, C. (1984). Complementation and genetic inactivation: two alternative mechanisms leading to prototrophy in diploid bacterial clones. Molecular and General Genetics 196: 488–493.
Article PubMed Google Scholar
Olsen, G.J., Woese, C.R. (1997). Archaeal genomics: An overview. Cell 89: 991–994. (An excellent review of the major types of prokaryotes.)
Shapiro, L. (1993). Protein localization and asymmetry in the bacterial cell. Cell 73: 841–855. (Although the main text of this review article is not relevant to this chapter, the article begins with an excellent review of cell division processes in bacteria and yeast.)
Download references
Author information
Authors and affiliations.
Department of Microbiology, Arizona State University, Tempe, AZ, 85287-2701, USA
Edward A. Birge
You can also search for this author in PubMed Google Scholar
Rights and permissions
Reprints and permissions
Copyright information
© 2000 Springer Science+Business Media New York
About this chapter
Birge, E.A. (2000). Fundamentals of Bacterial and Viral Genetics. In: Bacterial and Bacteriophage Genetics. Springer, New York, NY. https://doi.org/10.1007/978-1-4757-3258-0_1
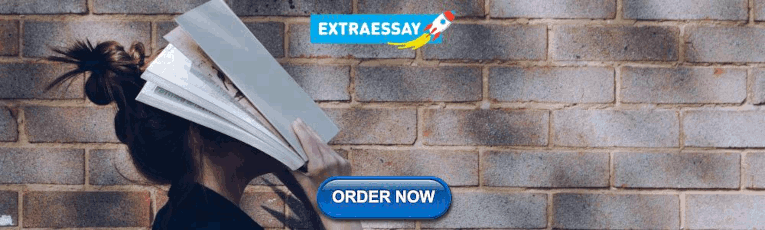
Download citation
DOI : https://doi.org/10.1007/978-1-4757-3258-0_1
Publisher Name : Springer, New York, NY
Print ISBN : 978-1-4757-3260-3
Online ISBN : 978-1-4757-3258-0
eBook Packages : Springer Book Archive
Share this chapter
Anyone you share the following link with will be able to read this content:
Sorry, a shareable link is not currently available for this article.
Provided by the Springer Nature SharedIt content-sharing initiative
- Publish with us
Policies and ethics
- Find a journal
- Track your research
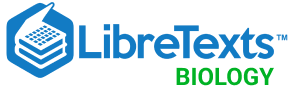
- school Campus Bookshelves
- menu_book Bookshelves
- perm_media Learning Objects
- login Login
- how_to_reg Request Instructor Account
- hub Instructor Commons
- Download Page (PDF)
- Download Full Book (PDF)
- Periodic Table
- Physics Constants
- Scientific Calculator
- Reference & Cite
- Tools expand_more
- Readability
selected template will load here
This action is not available.
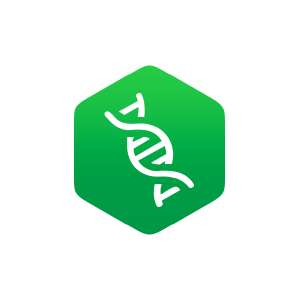
3: Bacterial Genetics
- Last updated
- Save as PDF
- Page ID 3149
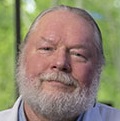
- Gary Kaiser
- Community College of Baltimore Country (Cantonsville)
- 3.1: Horizontal Gene Transfer in Bacteria Horizontal gene transfer enables bacteria to respond and adapt to their environment much more rapidly by acquiring large DNA sequences from another bacterium in a single transfer. Horizontal gene transfer is a process in which an organism transfers genetic material to another organism that is not its offspring. Mechanisms of bacterial horizontal gene transfer include transformation, transduction, and conjugation.
- 3.2: Bacterial Quorum Sensing, Pathogenicity Islands, and Secretion Systems (Injectosomes) Pathogenicity is the ability of a microbe to cause disease and inflict damage upon its host; virulence is the degree of pathogenicity within a group or species of microbes. The pathogenicity of an organism is determined by its virulence factors. Virulence factors enable that bacterium to colonize the host, resist body defenses, and harm the body. Most of the virulence factors are the products of quorum sensing genes.
- 3.3: Enzyme Regulation In living cells there are hundreds of different enzymes working together in a coordinated manner, and since cells neither synthesize nor break down more material than is required for normal metabolism and growth, precise enzyme regulation is required for turning metabolic reactions on and off. There is tremendous diversity in the mechanisms bacteria use to regulate enzyme synthesis and enzyme activity.
- 3.E: Bacterial Genetics (Exercises) These are homework exercises to accompany Kaiser's "Microbiology" TextMap. Microbiology is the study of microorganisms, which are defined as any microscopic organism that comprises either a single cell (unicellular), cell clusters or no cell at all (acellular). This includes eukaryotes, such as fungi and protists, and prokaryotes. Viruses and prions, though not strictly classed as living organisms, are also studied.
share this!
April 17, 2024
This article has been reviewed according to Science X's editorial process and policies . Editors have highlighted the following attributes while ensuring the content's credibility:
fact-checked
peer-reviewed publication
trusted source
Soil bacteria link their life strategies to soil conditions: Study
by US Department of Energy
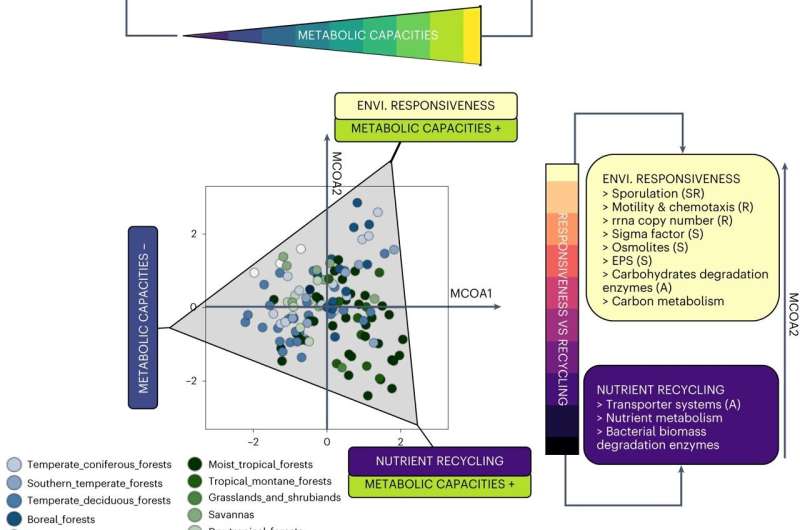
Soil bacteria help regulate the cycling of carbon and nutrients on Earth. Over time, these bacteria have evolved strategies that determine where they live, what they do, and how they deal with a changing environment. However, microbiologists do not fully understand how bacteria's genes relate to their life strategies.
By analyzing large DNA sequencing datasets from around the globe, researchers discovered a new way of categorizing the dominant life strategies of soil bacteria based on their genes. This technique allowed the researchers to link different life strategies with specific climate and soil conditions. Their paper is published in the journal Nature Microbiology .
Soil bacteria are crucial for planetary health, but they are hard to study because they are so diverse and invisible to the human eye. In their study, researchers used widely available gene sequence data to classify soil bacteria according to their life strategies. This makes it easier for researchers to predict how soils might respond to climate change or to engineer microbes with desirable life strategies. This research also helps solve the longstanding problem in biology of connecting gene sequences to the metabolism of bacteria and other life forms.
The research team categorized the life history strategies of soil bacteria using biological traits derived from metagenomic sequencing data. Next, the researchers used a mathematical technique to group the traits into life history strategies. Three main strategies emerged based on different genetic traits.
Some bacteria display a minimalist strategy, with small genomes that support basic metabolism and growth. Other bacteria have larger genomes, allowing them to extend their metabolic capacities. Bacteria with bigger genomes can react more effectively than bacteria with small genomes to changing environmental conditions and can more effectively recycle nutrients.
The most advantageous strategy in a given location depended on variables like soil pH, nitrogen availability, annual precipitation, and seasonality. Minimalist bacteria with small genomes were favored in pH-balanced soils exposed to drought and big seasonal swings, a strategy that the researchers related to stress tolerance.
Under dry, variable, and more acidic conditions, the preferred strategy was expanded metabolism allowing for rapid response to environmental changes . These bacteria resembled weedy plants that grow quickly after disturbances like drought or fire. The third major strategy—with expanded metabolism for nutrient cycling—was prominent among bacteria living in consistently wet, acidic soils . These bacteria are analogous to plants that out-compete their neighbors by overtopping them.
Journal information: Nature Microbiology
Provided by US Department of Energy
Explore further
Feedback to editors

Liquid droplets shape how cells respond to change, shows study
7 hours ago

Rice bran nanoparticles show promise as affordable and targeted anticancer agent

Advance in forensic fingerprint research provides new hope for cold cases
8 hours ago

How spicy does mustard get depending on the soil?

Electron videography captures moving dance between proteins and lipids

New findings shed light on how bella moths use poison to attract mates

AI tool creates 'synthetic' images of cells for enhanced microscopy analysis

Announcing the birth of QUIONE, a unique analog quantum processor

World's oases threatened by desertification, even as humans expand them
9 hours ago

NASA's Voyager 1 resumes sending engineering updates to Earth
Relevant physicsforums posts, the cass report (uk).
2 hours ago
Major Evolution in Action
If theres a 15% probability each month of getting a woman pregnant....
Apr 19, 2024
Can four legged animals drink from beneath their feet?
Apr 15, 2024
Mold in Plastic Water Bottles? What does it eat?
Apr 14, 2024
Dolphins don't breathe through their esophagus
More from Biology and Medical
Related Stories

Climate shapes life-history traits of abundant bacteria in Qinghai-Tibet plateau
Nov 24, 2023

Scientists discover how soil microbes survive in harsh desert environments
Apr 17, 2024

Genes identified that allow bacteria to thrive despite toxic heavy metal in soil
Mar 18, 2024

Researchers dish the dirt on soil microbes
May 17, 2019

Using bacteria to make lunar soil more fertile
Nov 10, 2023

Machine learning helps scientists identify the environmental preferences of microbes
Apr 28, 2023
Recommended for you

A small factor makes a big impact on genome editing
13 hours ago

The enemy within: How pathogens spread unrecognized in the body

Same species, different sizes: Rare evolution in action spotted in island bats
15 hours ago

Planning at multiple scales for healthy corals and communities
14 hours ago
Let us know if there is a problem with our content
Use this form if you have come across a typo, inaccuracy or would like to send an edit request for the content on this page. For general inquiries, please use our contact form . For general feedback, use the public comments section below (please adhere to guidelines ).
Please select the most appropriate category to facilitate processing of your request
Thank you for taking time to provide your feedback to the editors.
Your feedback is important to us. However, we do not guarantee individual replies due to the high volume of messages.
E-mail the story
Your email address is used only to let the recipient know who sent the email. Neither your address nor the recipient's address will be used for any other purpose. The information you enter will appear in your e-mail message and is not retained by Phys.org in any form.
Newsletter sign up
Get weekly and/or daily updates delivered to your inbox. You can unsubscribe at any time and we'll never share your details to third parties.
More information Privacy policy
Donate and enjoy an ad-free experience
We keep our content available to everyone. Consider supporting Science X's mission by getting a premium account.
E-mail newsletter
Academia.edu no longer supports Internet Explorer.
To browse Academia.edu and the wider internet faster and more securely, please take a few seconds to upgrade your browser .
Enter the email address you signed up with and we'll email you a reset link.
- We're Hiring!
- Help Center
Metagemonic analysis of bacterial genetic diversity in blood samples of Iranian healthy women

RELATED TOPICS
- We're Hiring!
- Help Center
- Find new research papers in:
- Health Sciences
- Earth Sciences
- Cognitive Science
- Mathematics
- Computer Science
- Academia ©2024
Research on bacteria in the mainstream of biology
Affiliation.
- 1 Department of Biology, Massachusetts Institute of Technology, Cambridge 02139.
- PMID: 3287618
- DOI: 10.1126/science.3287618
The study of the genetics, biochemistry, and physiology of bacteria during the last 40 years has provided the concepts and methods for the study of cells of all types at the molecular level. Although much is already known about the mechanisms bacteria use to regulate the expression of their genes, a great deal more remains to be discovered that will have relevance to both prokaryotic and eukaryotic cells. Similarly, the study in bacteria of the transactions of DNA, of the synthesis and function of the cell membrane, of differentiation, and of the interaction with eukaryotic cells will undoubtedly produce results of general importance. The advantages of using bacteria for these studies include their simple noncompartmented structure, the accessibility of their genetic material, and the possibility of correlating the expression of a gene in the intact cell with its expression in a system composed of highly purified components. Finally, the comparative study of a wide variety of microorganisms may result in a better understanding of the evolution of prokaryotes and eukaryotes and lead to a comprehensive theory of cell biology.
Publication types
- Research Support, U.S. Gov't, Non-P.H.S.
- Research Support, U.S. Gov't, P.H.S.
- Bacteria* / genetics
- Escherichia coli / genetics
- Gene Expression Regulation
- Models, Biological
- Models, Genetic
- Research Design*
Grants and funding
- AM-13894/AM/NIADDK NIH HHS/United States
- GM-07446/GM/NIGMS NIH HHS/United States

- Biological and Environmental Research
- BER Highlights
- Biological and Environmental Research Advisory Committee (BERAC)
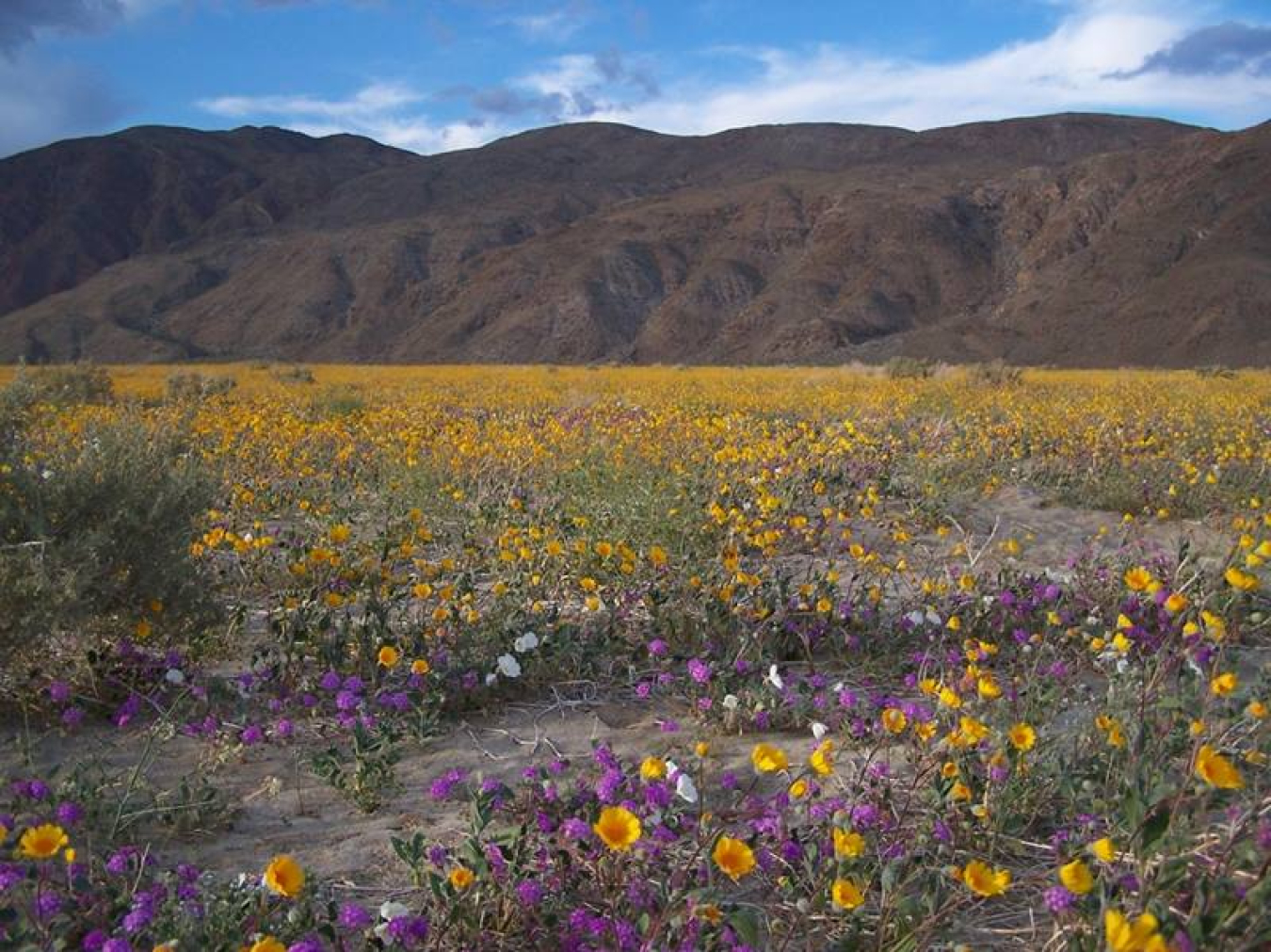
The Science
Soil bacteria help regulate the cycling of carbon and nutrients on Earth. Over time, these bacteria have evolved strategies that determine where they live, what they do, and how they deal with a changing environment. However, microbiologists do not fully understand how bacteria’s genes relate to their life strategies. By analyzing large DNA sequencing datasets from around the globe, researchers discovered a new way of categorizing the dominant life strategies of soil bacteria based on their genes. This technique allowed the researchers to link different life strategies with specific climate and soil conditions.
Soil bacteria are crucial for planetary health, but they are hard to study because they are so diverse and invisible to the human eye. Researchers used widely available gene sequence data to classify soil bacteria according to their life strategies. This makes it easier for researchers to predict how soils might respond to climate change or to engineer microbes with desirable life strategies. This research also helps solve the longstanding problem in biology of connecting gene sequences to the metabolism of bacteria and other life forms.
The research team categorized the life history strategies of soil bacteria using biological traits derived from metagenomic sequencing data. Next, the researchers used a mathematical technique to group the traits into life history strategies. Three main strategies emerged based on different genetic traits.
Some bacteria display a minimalist strategy, with small genomes that support basic metabolism and growth. Other bacteria have larger genomes, allowing them to extend their metabolic capacities. Bacteria with bigger genomes can react more effectively than bacteria with small genomes to changing environmental conditions and can more effectively recycle nutrients. The most advantageous strategy in a given location depended on variables like soil pH, nitrogen availability, annual precipitation, and seasonality. Minimalist bacteria with small genomes were favored in pH-balanced soils exposed to drought and big seasonal swings, a strategy that the researchers related to stress tolerance. Under dry, variable, and more acidic conditions, the preferred strategy was expanded metabolism allowing for rapid response to environmental changes. These bacteria resembled weedy plants that grow quickly after disturbances like drought or fire. The third major strategy—with expanded metabolism for nutrient cycling—was prominent among bacteria living in consistently wet, acidic soils. These bacteria are analogous to plants that out-compete their neighbors by overtopping them.
Gabin Piton University of California, Irvine and INRAE [email protected] Steven Allison University of California, Irvine [email protected]
This research was funded by the Department of Energy Office of Science, Biological and Environmental Research program, the European Research Council, and the U.K. Biotechnology and Biological Sciences Research Council Institute Strategic Program on Food, Microbiome and Health.
Publications
Piton, G. et al. , Life history strategies of soil bacterial communities across global terrestrial biomes. Nature Microbiology 8 , 2093–2102 (2023). [DOI: 10.1038/s41564-023-01465-0]
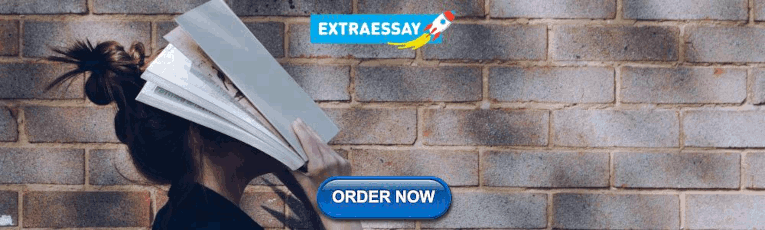
IMAGES
VIDEO
COMMENTS
Genetic changes also cannot explain the high reversion rates because for the bacteria to revert to susceptible phenotype, compensatory or back mutations are needed, and these are known to occur at a very low rate (51, 52). Adaptive resistance is the consequence of the presence of heritable phenotypic variations even in genetically identical ...
Author summary Escherichia coli is a usually harmless bacteria typically found in the human gut. However, certain strains can cause severe bloodstream infections (BSIs) with high mortality rates, especially in older and fragile patients. To develop more effective prevention and treatment measures, understanding the genetic factors that determine a strain's capacity to cause infection is ...
Introduction. Escherichia coli (or E. coli) is a Gram-negative versatile bacterium, easily found and amenable to natural and random genetic alteration.There is a vast collection of sequenced E. coli genomes which exhibit different sizes and genomic diversity among commensal and pathogens, indicating a great assortment within the same bacterial species.
Section Information. The aim of the section "Microbial Genetics and Genomics" is to provide a platform for current research on archaea, bacteria, microbial eukaryotes, and viruses. We welcome studies that apply recent advances in genetics, genomics, transcriptomics, proteomics, metabolomics and computational biology to provide insights into ...
Pseudomonas aeruginosa is a versatile, adaptable bacterial species with widespread environmental occurrence, strong medical relevance, a diverse set of virulence genes and a multitude of intrinsic and possibly acquired antibiotic resistance traits. Pseudomonas aeruginosa causes a wide variety of infections and has an epidemic-clonal population ...
This medical problem stimulated research in bacterial genetics which revealed that horizontal gene transfer is involved in some of the genetic variations causing resistance to antibiotics. ... Papers on Bacterial Genetics. Little, Brown and Co.; New York, NY, USA: 1960. pp. 224-229. [Google Scholar] 10.
coli and Bacillus subtilis have dominated research because of their genetic tractability and culturing ease. Many of the more strangely shaped bacteria proved unculturable, or their original strains were lost. ... Cohen SN, Chang ACY, Hsu L. Nonchromosomal antibiotic resistance in bacteria: genetic transformation of Escherichia coli by R-factor ...
Abstract. The availability of hundreds of bacterial genome sequences has altered the study of bacterial pathogenesis, affecting both design of experiments and analysis of results. Comparative genomics and genomic tools have been used to identify virulence factors and genes involved in environmental persistence of pathogens.
This Special Issue gathers eight research papers related to microbial genetics and evolu-tion. A crucial step in investigating the evolution of an organism and its genome is to define. its ...
This Special Issue of Microorganisms aims to expand the current state of the art regarding all the areas of bacterial genetics, not limited to those mentioned above. Research articles, reviews, and short communications concerning the current challenges of bacterial genetics are of interest. Prof. Dr. Renato Fani. Prof. Dr. Marco Bazzicalupo.
Thus, this system provides a useful tool for single-copy complementation at an episomal site for research in bacterial genetics and microbial pathogenesis. Furthermore, these vectors could also be used for the introduction of foreign genes for use in biotechnology applications, vaccine development, or gene expression and gene fusion constructs.
efore beginning the formal study of the genetics of bacteria and their viruses, it is important to have a clear understanding of the fundamentals of microbial cell structure and of basic viral processes. This chapter briefly reviews cell anatomy as well as genetic terminology and processes with respect to bacteria, yeast, and viruses.
In 1987, the New York Times Magazine characterized the Human Genome Project as the "biggest, costliest, most provocative biomedical research project in history." 2 But in the years between the ...
Microbial genetics is still in a phase of expansion and the splitting of the subject this year has somewhat relieved the reviewer's labours. The present paper attempts to cover the developments in the genetics of bacteria which have taken place in the years 1955 and 1956, i.e., subsequent to the publication of the previous review by Zelle (181). Work on phage will be considered only insofar as ...
The first chapter on microbial genetics started by George W. Beadle (1903-1989) and Edward L. Tatum (1909-1975) while investigating genetics of tryptophan metabolism and nicotinic acid ...
Rice (Oryza sativa L.) is one of the most important food crops grown in various agro-climatic conditions throughout the world.More than 90% of the world's rice is produced and consumed in Southeast Asia and tropical Latin America. In India, rice is grown on about 4.4 × 10 7 hm 2 field and provides food for more than 75% of the population. To meet the growing demand of nearly 5.0 billion ...
Abstract. The study of the genetics, biochemistry, and physiology of bacteria during the last 40 years has provided the concepts and methods for the study of cells of all types at the molecular level. Although much is already known about the mechanisms bacteria use to regulate the expression of their genes, a great deal more remains to be ...
Neutral Theory, Microbial Practice: Challenges in Bacterial Population Genetics. Mol Biol Evol2018 Jun 1;35 (6):1338-1347. doi: 10.1093/molbev/msy078. Eduardo P C Rocha. Microbial Evolutionary Genomics, Institut Pasteur, Paris, France. CNRS, UMR3525, Paris, France. 29684183. 10.1093/molbev/msy078. I detail four major open problems in microbial ...
Bacterial genetics is the subfield of genetics devoted to the study of bacteria. Bacterial genetics are subtly different from eukaryotic genetics, however bacteria still serve as a good model for animal genetic studies. One of the major distinctions between bacterial and eukaryotic genetics stems from the bacteria's lack of membrane-bound ...
DOI: 10.1038/s41564-023-01465-. Soil bacteria help regulate the cycling of carbon and nutrients on Earth. Over time, these bacteria have evolved strategies that determine where they live, what ...
Academia.edu is a platform for academics to share research papers. Metagemonic analysis of bacterial genetic diversity in blood samples of Iranian healthy women ... Metagemonic analysis of bacterial genetic diversity in blood samples of Iranian healthy women. 15 Pages.
Shifting Focus: Early Work on Bacterial Transformation, 1928-1940; DNA as the "Stuff of Genes": The Discovery of the Transforming Principle, 1940-1944 ... Genetic Remove constraint Subject: Recombination, Genetic Periodical Experimental Cell Research ...
Abstract. The study of the genetics, biochemistry, and physiology of bacteria during the last 40 years has provided the concepts and methods for the study of cells of all types at the molecular level. Although much is already known about the mechanisms bacteria use to regulate the expression of their genes, a great deal more remains to be ...
April 17, 2024. Biological and Environmental Research. Soil Bacteria Link their Life Strategies to Soil Conditions. Like desert wildflowers that bloom after rain, soil bacteria have evolved life strategies that determine when and where they grow. Having a small, streamlined genome may help some bacteria survive in dry soils.