share this!
September 4, 2020 report
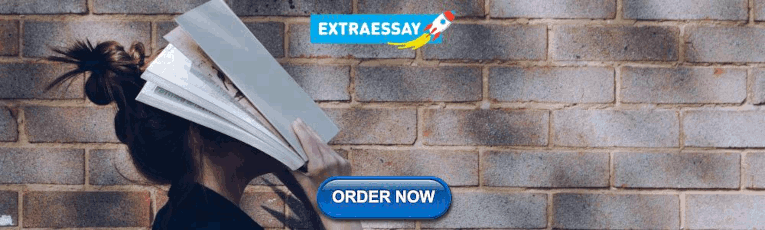
New insights into the global silicon cycle
by Bob Yirka , Phys.org
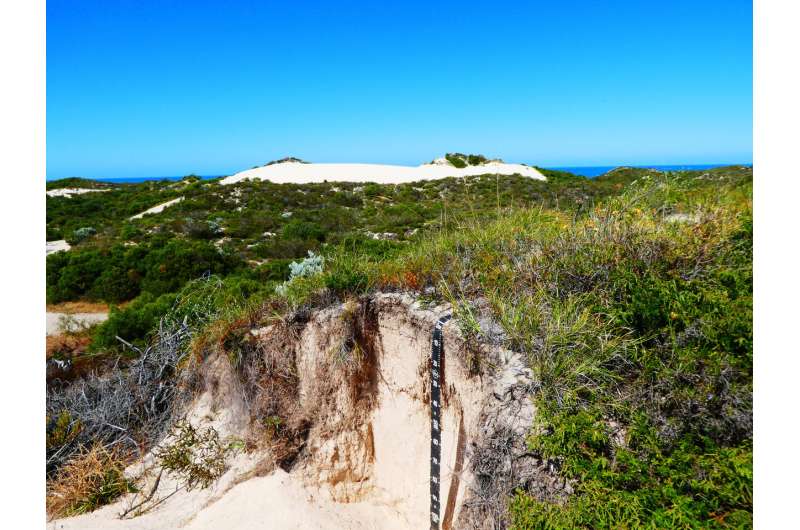
An international team of researchers has learned more about the global silicon cycle by studying ancient soil samples in Australia. In their paper published in the journal Science , the group describes their study of soil of different ages and what they learned about it. Joanna Carey with Babson College, has published a Perspective piece in the same journal issue outlining the connection between the carbon and silicon cycle and the work done by the team in this new effort.
Silicon is the second-most abundant element in Earth's crust and it plays a vital role in plant life, both on land and in the sea. Silicon is used by plants in tissue building, which helps to ward off herbivorous animals. In the ocean, phytoplankton consume enormous amounts of silicon ; they get a constant supply courtesy of rivers and streams. And silicon winds up in rivers and streams due to erosion of silicon-containing rocks. Land plants also use silicon. They get it from the soil. In this new effort, the researchers began by noting that the terrestrial biogeochemical cycling of silicon (how it moves from plants back to the soil and then into plants again) is poorly understood. To gain a better understanding of how it works, they ventured to a part of Western Australia that, unlike other parts of the world, has not been impacted by Pleistocene glaciations. The soil there gave the researchers a look at the silicon cycle going back 2 million years.
In analyzing soil samples from multiple depths, the researchers were able to compare old and comparatively new soil. They determined that as the soil aged, it contained less silicon—it had become depleted likely by rainwater carrying it away. But the newer soil above it had more silicon as rock broke down, releasing its silicon content. The researchers suggest their findings indicate that silicon cycling shifts from geochemical to biological control over time, with plants as the regulating element. They further suggest that such cycling allows plants to absorb as much silicon as they need, even in soil that has been depleted in other ways. Carey adds that more knowledge about how the silicon cycle works should lead to a better understanding of the carbon cycle, because the two are linked in many ways.
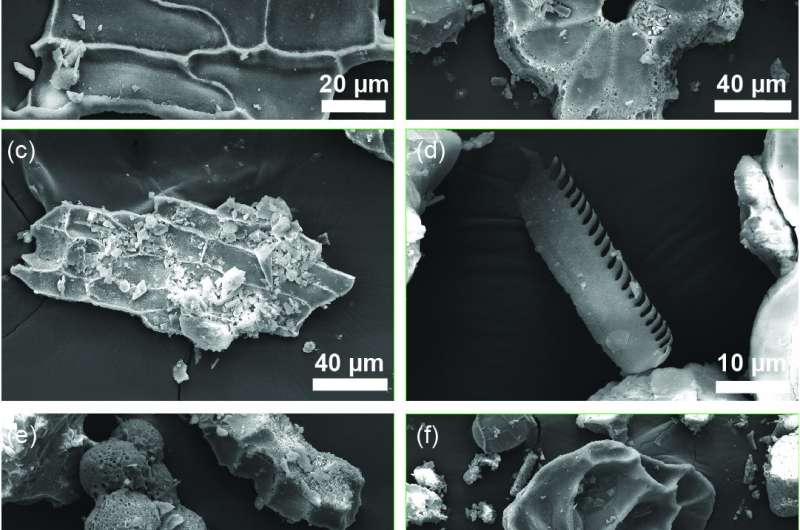
Joanna Carey. Soil age alters the global silicon cycle, Science (2020). DOI: 10.1126/science.abd9425
Journal information: Science
© 2020 Science X Network
Explore further
Feedback to editors

Researchers establish commercially viable process for manufacturing with promising new class of metals
34 minutes ago

Chimps shown to learn and improve tool-using skills even as adults

Advanced experimental setup expands the hunt for hidden dark matter particles
2 hours ago

New research confirms that Beethoven had lead poisoning—but it didn't kill him

How NASA's Roman mission will hunt for primordial black holes

Geologists reveal mysterious and diverse volcanism in lunar Apollo Basin, Chang'e-6 landing site
3 hours ago

Using algorithms to decode the complex phonetic alphabet of sperm whales

Researchers 'unzip' 2D materials with lasers

How a 'conductor' makes sense of chaos in early mouse embryos
4 hours ago

Decoding development: mRNA's role in embryo formation
Relevant physicsforums posts, the secrets of prof. verschure's rosetta stones.
May 2, 2024
Large eruption at Ruang volcano, Indonesia
Tidal friction and global warming.
Apr 20, 2024
Iceland warming up again - quakes swarming
Apr 18, 2024
M 4.8 - Whitehouse Station, New Jersey, US
Apr 6, 2024
Major Earthquakes - 7.4 (7.2) Mag and 6.4 Mag near Hualien, Taiwan
Apr 5, 2024
More from Earth Sciences
Related Stories

Perovskite mineral supports solar-energy sustainability
Aug 3, 2020

Silicon: An important element in rice production
Apr 28, 2015

Adding silicon to soil to strengthen plant defenses
Aug 15, 2017

Freeze-dried soil is more suitable for studying soil reactive nitrogen gas emissions
Mar 5, 2020

Silicon 'plant stones' for strong rice: Fertilizing and recycling Si in Vietnamese fields
Oct 1, 2015

Planting non-native trees accelerates the release of carbon back into the atmosphere
Jun 17, 2020
Recommended for you

Discharge of scrubber water into the Baltic Sea is responsible for hundreds of millions in costs
10 hours ago

Computer models show heat waves in north Pacific may be due to China reducing aerosols
6 hours ago

Study identifies early warning signals for the end of the African humid period

Researchers show that slow-moving earthquakes are controlled by rock permeability

Earthquakes are moving northeast in Midland Basin of Texas, scientists find
23 hours ago

Researchers find Northern Hemisphere glaciation enhances orbital- and millennial-scale Asian winter monsoon variability
May 6, 2024
Let us know if there is a problem with our content
Use this form if you have come across a typo, inaccuracy or would like to send an edit request for the content on this page. For general inquiries, please use our contact form . For general feedback, use the public comments section below (please adhere to guidelines ).
Please select the most appropriate category to facilitate processing of your request
Thank you for taking time to provide your feedback to the editors.
Your feedback is important to us. However, we do not guarantee individual replies due to the high volume of messages.
E-mail the story
Your email address is used only to let the recipient know who sent the email. Neither your address nor the recipient's address will be used for any other purpose. The information you enter will appear in your e-mail message and is not retained by Phys.org in any form.
Newsletter sign up
Get weekly and/or daily updates delivered to your inbox. You can unsubscribe at any time and we'll never share your details to third parties.
More information Privacy policy
Donate and enjoy an ad-free experience
We keep our content available to everyone. Consider supporting Science X's mission by getting a premium account.
E-mail newsletter
University of Helsinki
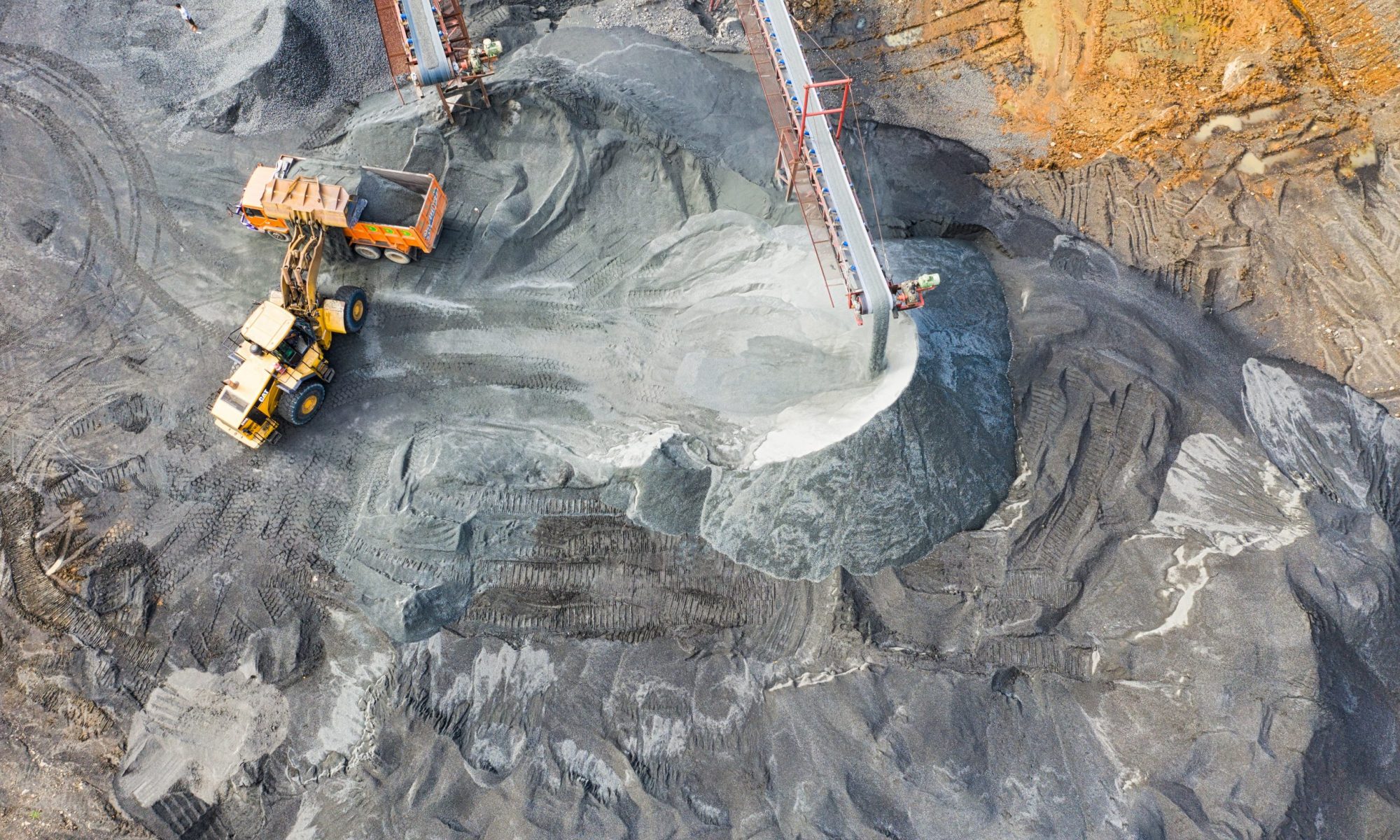
Dirty side of green technology
Geographies of Inequalities 2021
Exploring the Negative Impacts of Mining Minerals
Lithium production, its negative impacts and distribution globally, text by nella koivula.
Global lithium production
Lithium is one of the most important minerals used for green technologies and therefore an important mineral to consider regarding sustainable development of the future. Due to the shift from fossil fuel vehicles to electric vehicles, the demand for lithium is estimated to explode in the future. According to Roskill, in 2019, rechargeable batteries that are used in most green technologies, accounted for 54% of total lithium demand (Roskill, 2020). Statista reports that an estimated total of 82,000 metric tons of lithium was produced globally in 2020 while ten years earlier production was just 28,000 metric tons (Garside, 2021). Similarly, according to Roskill lithium consumption for rechargeable batteries increased 14% from 2007 to 2017 (Roskill, 2020).

The rapidly growing demand for lithium-ion batteries raises the question whether there is enough lithium in the world for the transition to green technologies? Some argue that the global resources of lithium will not be enough for the future demand and therefore, there is a need to find solutions for the recycling of materials (Hunt, 2015; Willuhn, 2020). Lithium demand is expected to grow 5 times higher in the next decade and due to this the EU has added lithium to the list of critical metals (Kriittiset materiaalit, 2021). This massive demand for lithium is consequently reflected on lithium prices which are estimated to rise in coming years (Mining.com, 2021).
Currently the biggest lithium producers are Australia, Chile, China, Argentina, Brazil, Zimbabwe and Portugal (see map below)(Garside, 2021). There are two main ways of lithium extraction: from hard rock deposits and from salt lake deposits. In South America lithium carbonate is extracted from salt lake deposits from where it is evaporated and washed with polyvinyl chloride in shallow ponds (Garrett, 2004 as cited in Wanger, 2011). Lithium extraction from salt lake deposits demands a lot of water which can be costly for the environment and local communities because these areas already suffer from drought. South America’s lithium boom has potential to bring economic growth and development for the local communities and reduce poverty. However, profits rarely go back to the local communities and increasing mining in these areas can be costly for the environment and for the livelihoods of indigenous people.

More than half of the world’s lithium production comes from South America, mainly from Argentina and Chile (Alves, 2021). Currently Chile is the lithium-richest country in the world with 43.7 percent share of total global reserves. While Chile and Argentina have seen economic growth from the mining sector, Bolivia, even with estimated world’s largest lithium reserves, is still one of Latin America’s poorest nations. Bolivia has approximately 9 million tons of lithium reserves, but they have not yet been exploited or used commercially due to high altitude and lack of appropriate infrastructure. However, the government is eager to jump into the bandwagon of mineral production and make Bolivia one of the world’s top-producing nations. (Bloomberg News, 2018.)
Lithium can also be mined from pegmatite ores from where it is processed of spodumene, the main lithium carrier in magmatic rocks (Bridge 2004 as cited in Wanger, 2011). This is done in, for example, Zimbabwe and Canada. Also, the premeditated mine in Finland would extract lithium from hard rock deposits. Similarly to salt flat extraction, lithium mining from pegmatite ores is costly for the environment because it causes physical land rearrangements and waste products which can damage soil, biodiversity and existing ecosystems, (Bridge 2004 as cited in Wanger, 2011).
From the lithium-richest countries lithium is often shipped to China and South Korea to be used in smartphones and green technology. China is an important manufacturer, consumer and supplier of lithium batteries (The Wall Street Journal, 2021). In 2020 China produced only 11% of the raw material but refined 60% of the lithium globally (Kriittiset materiaalit, 2021; IEEE, 2012). Therefore, China is highly dependent on imports and it is the world’s largest lithium consumer accounting for approximately 50% of the global total (S&P Global Market Intelligence, 2021).
As can be seen from the map, global lithium production is currently distributed quite unevenly. From a domestic perspective, Finland is aiming to start producing lithium self-sufficiently in the coming years.

Case of South America: negative impacts of lithium extraction from salt flats
South America is one of the most important geographical areas for lithium production. Chile, Argentina and Bolivia form a ‘lithium triangle’ which is responsible for the vast majority of global lithium production (Alves, 2021). Lithium is found in the salt flats in high mountains where lithium carbonate is produced by evaporation and washing (Garrett, 2004 as cited in Wanger, 2011). Multiple studies have shown that mining poses severe reproductive social and environmental threats that may have long-term consequences to biodiversity and the livelihoods of local communities (Sonter & Ali & Watson, 2018; Mancini & Sala, 2018). For example, one of the world’s largest lithium extraction site, Salar de Atacama in Chile has seen environmental degradation of salt flats, human settlements and national reserves in terms of vegetation decline, elevating daytime temperatures, decrease of soil moisture and increasing drought conditions during the past 20 years (Liu & Agusdinata & Myint, 2019). Moreover, negative side effects of mining include for example air pollution and water shortages because lithium extraction from salt flats – in an already arid environment – demands a lot of water (DW, 2018).
Water shortage and competition over water between local communities and mining companies can cause major problems. For example, in Chile there have been conflicts with mining companies and indigenous people over water resources in Atacama salt flats (Sherwood, 2018). In Chile, water resources and management are completely privatized. Mining companies such as SQM and Albemarle own the rights to use water in the region and the state has been reluctant to ban water extraction for mining (Sherwood, 2018). Since Atacama desert is one of the driest regions on Earth and lithium extraction from salt flats uses a lot of water, lithium mining has significant negative impacts on the water reserves, indigenous people and vulnerable species in the surroundings (DW, 2018) Lithium extraction can dry out rivers, streams and wetlands, contaminate drinking water and even damage entire ecosystems (DW, 2018). For example, the ecosystem of flamingos is endangered because of an increasing amount of microbial biomass and toxic cyanobacteria due to mining (Bauld 1981 as cited in Wanger 2011; Gutierrez & Navedo & Soriano-Redondo, 2018).
In addition, lithium extraction in South America can be costly for the local communities. As mentioned before, competition over water and degradation of the environment can disturb livelihoods of local communities. There is a so-called resource paradox: on the one hand lithium production can bring wealth, economic growth and job opportunities but on the other hand this may be costly when the mineral reserves finally run out and there are no longer environmental resources or jobs for the local communities (NowThis World, 2017).
Case of Finland: Domestic lithium production in Kaustinen
Finland is attempting to start a domestic lithium production in the following years (see map below). Mining company Keliber is planning Europe’s largest lithium mine in Kaustinen with which it aims to answer the rapidly growing demand of lithium in the era of renewable energy, electric vehicles and green technology (Keliber, 2021). Keliber is owned by Finnish investment companies, private companies and leading international mining company Sibanye-Stillwater and Norwegian Nordin Mining ASA. The estimated time for mine to start operating is in 2024. In addition, Keliber has applied permission for a lithium chemical plant in the mining area of Kokkola where lithium can be refined and developed into lithium hydroxide that can be used for lithium batteries for green technologies (Keliber, 2021).

Lithium mine in Kaustinen would be internationally remarkable since it would be the first of its kind in Europe. According to Keliber the mining site would last for approximately 13 years in large scale production and it has resources of approximately 11 million tons of lithium. Moreover, it is estimated to take charge of 3-5% of global lithium production (Keliber, 2021). According to some calculations this would be enough for approximately 200 000 – 250 000 electric cars (Yle Uutiset, 2019). Therefore, could lithium mine in Kaustinen be a way to shorten the supply chain of lithium and allow Finland to be better involved in the global responsibility for green technology mineral production? A key question is whether the amount of lithium that can be produced in 13 years and its capacity to produce batteries for green technology is enough to overcome the negative environmental effects that the mine could cause?
According to Environmental Impact Assessment, the mine is assessed to cause relatively little damage for the environment (Keliber, 2021). However, there would be some impacts on land and soil, ground and surface waters, vegetation, organisms and biodiversity. It can also cause damage for habitats of some species which is why Keliber is considering finding new habitats for some endangered species such as moor frogs and golden eagles. (Keliber, 2021.) In addition, there are concerns about the negative effects on fish and fishing in the surrounding areas (Yle Uutiset, 2020). The upside is that the mines would be far away from residential areas which decreases the risk of negative impacts on the surrounding communities (Keliber, 2021).
After the Talvivaara incident, negative impacts of mining on surrounding waters is a common concern in Finland. According to EIA, mining operations in Kaustinen are not estimated to have significant effects on the condition or acidity of the waters. However, even though ELY Centre estimated the effects to the surface waters as small, waste waters and by-products is an issue that needs careful assessment. (Keliber, 2021). Wastewaters were initially aimed to be disposed of by evaporation but with technological advantages wastewaters can be more efficiently disposed of by using EWT (electric-chemical processing) and DAF (microflotation). Subsequently remaining wastewater will be sanitized in Hopeakivenlahti wastewater treatment plant where it will be released back to the ocean. Wastewaters will contain small amounts of lithium, but the concentration of minerals should be well below harmful level and once it mixes with sea water it should not harm any species that are exposed to it long-term (Keliber, 2021). In addition, Keliber aims to utilize waste rocks as much as possible in construction of Kokkola harbour and mining areas by exploiting cleantech processes in collaboration with Outotec. (Keliber, 2021). Keliber’s objective is to rehabilitate mining areas into biodiverse environments as quickly as possible by turning the pits into lakes and using the piled soil for landscaping and vegetation purposes. (Keliber, 2021).
Although Keliber has potential to respond to domestic and European demand for green technology, will Finland remain only as a producer of raw materials for green technology batteries? Finland has the capacity to shorten the supply chain which is also what Keliber is aiming for with the development of chemical plants in the Kokkola region where lithium can be used for batteries. Therefore, the lithium mine in Kaustinen has a lot of potential to increase Finland’s share of global lithium production for green technologies.
Overall, the sustainability of mining is controversial because mines are located unevenly, often in developing countries even though most of those benefiting from green technologies are in the Western world. Therefore, most of the environmental and social spillover effects of the mining industry accumulate to developing countries while some societies benefit from the ability to transform into carbon-free lifestyles without negative effects on their environment. Thus, it is important to consider how much increasing demand of minerals and metals for the green technology transition can lead to exploitation of resources and negative social, environmental and economic spillover effects in certain parts of the world. This raises a question: How can we take more global responsibility for the negative spillover effects of the mining industry to ensure sustainable development equally? As mentioned before, domestic lithium production in Finland could be one way to increase Finland’s and Europe’s responsibility for mining for green technologies.
Alves, B. (2021) Lithium in South America — Statistics and Facts. Statista. Retrieved from https://www.statista.com/topics/5894/lithium-in-south-america/
Benchmark Mineral Intelligence (2020) Lithium in 2020: Prices, Coronavirus and Tesla. Retrieved from https://www.benchmarkminerals.com/membership/lithium-in-2020-prices-coronavirus-and-tesla/
Bloomberg News (2018) Big lithium riches lure first-time miner to Bolivia’s salt flat. Retrieved from http://www.mining.com/web/big-lithium-riches-lure-first-time-miner-bolivias-salt-flat/
DW (2018) Chile’s lithium – blessing or curse? Retrieved from https://www.dw.com/cda/en/chiles-lithium-blessing-or-curse/a-43721539
Elinkeino-, liikenne- ja ympäristökeskus (2020) Yhteysviranomaisen perusteltu päätelmä litiumkemiantehdas -hankkeen ympäristövaikutusten arviontiselostuksesta.
Garside, M. (2021) Global lithium mine production 2010-2020. Statista. Retrieved from https://www.statista.com/statistics/606684/world-production-of-lithium/
Garside, M. (2021) Major countries in worldwide lithium mine production from 2010 to 2020. Statista. Retrieved from https://www.statista.com/statistics/268789/countries-with-the-largest-production-output-of-lithium/
Gutierrez, J. S. & Navedo, J. G. & Soriano-Redondo, A. (2018) Chilean Atacama site imperilled by lithium mining. Nature. Retrieved from https://www.nature.com/articles/d41586-018-05233-7
Helsingin Sanomat (2021) Sähköautojen räjähdysmäisesti kasvanut suosio on johtanut litium-pulaan – Akkumetallin hinta on jo noussut vauhdilla, ja pian sen hinta nousee pilviin. Retrieved from https://www.hs.fi/paivanlehti/22032021/art-2000007872976.html
Hunt, T. (2015) Is There Enough Lithium to Maintain the Growth of the Lithium-Ion Battery Market? Gtm. Retrieved from https://www.greentechmedia.com/articles/read/is-there-enough-lithium-to-maintain-the-growth-of-the-lithium-ion-battery-m
IEEE International Symposium on Sustainable Systems and Technology (2012) Prediction of various discarded lithium batteries in China. DOI: 10.1109/ISSST.2012.6228021
Kaunda, R. B. (2020) Potential environmental impacts of lithium mining, Journal of Energy & Natural Resources Law, 38:3, 237-244, DOI: 10.1080/02646811.2020.1754596
Keliber (2021) Lithium markets. Retrieved from https://www.keliber.fi/en/production-and-products/lithium-markets/ . Cited 24.4.2021.
Keliber (2021) Mineral resources and ore reserves. Retrieved from https://www.keliber.fi/en/geology/mineral-resources-and-ore-reserves/- Cited 24.4.2021.
Keliber (2021) YVA. Retrieved from https://www.keliber.fi/ajankohtaista/raportit-ja-julkaisut/yva/ Cited 24.4.2021.
Keliber (2021) Keliber päivitti Kokkolan kemiantehtaan prosessia ja YVA-selostusta. Retrieved from https://www.keliber.fi/ajankohtaista/tiedotteet-ja-julkaisut/81C971D5188FA114/ . Cited 24.4.2021.
Keliber (2021) We respect people and the environment. Retrieved from https://www.keliber.fi/en/sustainability/we-respect-people-and-the-environment/ . Cited 24.4.2021.
Kriittiset materiaalit (2021) Litium. Retrieved from https://www.kriittisetmateriaalit.fi/litium/ .
Liu, W. & Agusdinata, D. & Myint, S. (2019) Spatiotemporal patterns of lithium mining and environmental degradation in the Atacama Salt Flat, Chile. International Journal of Applied Earth Observation and Geoinformation. 80. 145-156. DOI: 10.1016/j.jag.2019.04.016
Mancini, L. & Sala, S. (2018). Social impact assessment in the mining sector: Review and comparison of findicators frameworks. Resources Policy 57, https://doi.org/10.1016/j.resourpol.2018.02.002
Mining.com (2021) Lithium prices continue to soar — up 88% in 2021. Retrieved from https://www.mining.com/lithium-prices-continue-to-soar-up-88-in-2021/
NowThis World (2017) South America’s Lithium Boom: A Blessing Or A Curse? Retrieved from https://www.youtube.com/watch?v=AsxjjLbn28Q
Roskill (2020) Lithium: Outlook to 2030, 17th Edition. Retrieved from https://roskill.com/market-report/lithium/ .
Sherwood, D. (2018) In Chilean desert, global thirst for lithium is fueling a ‘water war’. Reuters. Retrieve from https://www.reuters.com/article/us-chile-lithium-water/in-chilean-desert-global-thirst-for-lithium-is-fueling-a-water-war-idUSKCN1LE16T
S&P Global Market Intelligence (2021) Essential Energy Insights. Retrieved from https://www.spglobal.com/marketintelligence/en/news-insights/blog/essential-energy-insights-march-2021
Sonter LJ, Ali SH, Watson JEM. 2018 Mining and biodiversity: key issues and research needs in conservation science. Proc. R. Soc. B 285: 20181926. http://dx.doi.org/10.1098/rspb.2018.1926
The Wall Street Journal (2021) Shift to Electric Vehicles Spurs Bid to Make More Batteries in U.S. Retrieved from https://www.wsj.com/articles/u-s-mounts-a-charge-to-take-on-china- the-king-of-electric-vehicle-batteries-11611658235
Wanger, T. (2011) The Lithium future — resources, recycling, and the environment. Conservation Letters. DOI: 10.1111/j.1755-263X.2011.00166.x
Willuhn, M. (2020) How long will the lithium supply last? Pv magazine. Retrieved from https://www.pv-magazine.com/2020/09/15/how-long-will-the-lithium-supply-last/
From outsourced to insourced: sustainable development goals, green technology transformations and critical minerals in the European Union
Text by niina asikanius.
In the face of ever-growing environmental degradation and pressures to seek transformative enough solutions to sustainability issues, the European Union has put forth action plans and strategies to seek sustainable pathways to growth. Already in 2010, in the wake of the 2008 financial crisis, the EU introduced the Europe 2020 strategy, where economic and social progress as well as structural changes were ambitiously being planned to carry out through three mutually reinforcing priorities: smart growth, sustainable growth and inclusive growth. This strategy also includes seven flagship initiatives, one of which is “Resource efficient Europe” where focus is put on the decoupling of economic growth from the use of resources, shift towards a low carbon economy as well as increasing the use of renewable energy sources and promoting energy efficiency and modernizing the transport sector. (European Commission1, 2010, 3-4). A newer strategy, the European Green Deal, has been designed to further tackle climate and environment related challenges. The overall aim of this deal is to create a growth strategy that will transform the EU into a fair and prosperous society by 2050. Visions for the strategy include resource-efficiency, competitive economy as well as a promise of no net greenhouse gas emissions, continuing along earlier ideas of decoupling economic growth from resource use. The Green deal is also an integral part of the European Commission’s strategy to implement not only the United Nations sustainable development goals but also the United Nations 2030 Agenda among other political guidelines. In order to reach these ambitious climate neutrality and sustainability goals, the EC intends to mobilize industry, where resource extraction and processing of materials cause half of greenhouse gas emissions and almost all of biodiversity loss and water stress, and shift from a linear pattern of production and dependence of extracted new materials to a sustainable model of inclusive growth. The EC has also recognized the importance of access to resources which is a strategic security question for Europe and the Green Deal. Critical raw materials are recognized as necessary materials for clean technologies and other applications. Of importance seems to be diversification of both primary and secondary sources for the abovementioned visions to become reality (European Commission 2, 2019). In addition, the EC has also proposed to modernize EU legislation on batteries to promote sustainable practices and its Circular Economy Action Plan. The proposal includes aspects such as sustainable life cycles for batteries placed in the EU market and mandatory requirements which all batteries need to meet in order to have the chance to be placed in the EU market (European Commission3, 2020).
Critical raw materials: lithium
With this new focus on transformations also comes the question of what the best tools are to execute necessary change. One sector that has gained growing attention is the mineral and mining industry because of its relation to green technology transitions, particularly the battery industry. According to the EU, batteries are a key technology in the transition to climate neutrality, and even to circular economy as well as sustainable mobility and in cutting down pollution. Because of the permeating role of batteries in our lives, the demand for them is projected to grow rapidly. EU also seems to acknowledge that increasing deployment of batteries in everyday functions means that the batteries market is a strategic one at the global level, and because of this the union, in order to guarantee a smooth green transition for the European region, wants to take a more active role in the sustainable production, deployment and waste management of all batteries placed in the EU market (European Commission4, 2020).

What will be focused on next are one of the key elements of battery technology: lithium. OECD predicts that extraction of metals and the processing of key metals are going to at least double between 2017-2060. This is driven by the growing scale of materials use (OECD , 2019, 16). The global use of lithium has increased, along with other metals. Some link this increase in usage with China’s economic reforms and development. China also dominates the lithium product manufacturing industry due to its manufacturing capacity and cheap products. In addition, Chinese manufacturing companies Tianqi and Ganfeng Lithium control almost half of the world’s lithium production. Because of this dominant market role as well as previous limitations of lithium export quotas, the reliance on China’s operations in lithium production could mean potential supply security issues (Kavanagh, Keohane, Garcia Cabellos, Lloyd, & Cleary, 2018, 2-3) Lithium, with cobalt and natural graphite, have been placed on the list of critical raw materials by the EU. In addition, the EU only produces 1% of all battery raw materials and only has 8% share of the supply of battery materials. However, supply of lithium isn’t expected to be a major issue in battery supply chain in short or medium term, but for the long term an increase from current low prices are thought to be necessary to support the development of new production capacity (Bobba, Carrara, Huisman, Mathieux, Pavel, 2020, 19-20). Currently, there are also gaps in the production chains of lithium in Europe. Lithium mined in Europe is still processed outside of the EU so vulnerabilities in the supply chain need addressing to avoid disruption to manufacturing processes (European Commission5, 2020). Circular economy practices are also crucial to develop and implement. If levels of reuse and recycling remain low, mineral depletion may become an issue. This issue is especially driven by the growing use of batteries in electric vehicles. According to some scenarios, even if lithium recycling was increased to 30%, the current estimated reserves would have been extracted by 2050 (de Blas, Mediavilla, Capellán-Pérez, & Duce, 2020, 13).
The complicated geographies of critical raw materials such as lithium then can create geopolitical risks as well as economic risks if smooth supply of materials is interrupted and markets can be manipulated by singular, dominant actors. For the EU this means that diversifying the materials supply is needed. This can be executed through secure trade agreements with third countries and economic diplomacy for lithium and other raw materials. Instead of focusing on improving supply risks in outsourced production chains, improving manufacturing opportunities in the EU is also an option. This would mean increase of mining, extraction and refining of key raw materials inside the region as well as the creation of a properly functioning ecosystem for battery manufacturing for local value chains to flourish but also to attract foreign investment. Other recommendations include better recycling activities, promotion of research and development investments as well as fostering international collaboration and standardization activities (Bobba, Carrara, Huisman, Mathieux, Pavel, 2020, 23). Somewhat in line with these recommendations, The EC has set its goals towards the increase of sustainable supply of raw materials in Europe by bringing together stakeholders along strategic value chains and industrial ecosystems (European Commission6). For example, new industrial alliances such as European Raw Materials Alliance and European Battery Alliance have been founded to mobilize public and private investment as well as improve EU resilience in rare earths value chain (European Commission5, 2020). So, the EU seems to have developed its intraregional strategies regarding critical raw materials to on one hand respond to supply risks and on the other hand to deal with economic importance of critical raw materials as well as sustainable futures.
EU’s raw materials strategies have also faced criticism for its lack of considerations of environmental and social impacts. The European Environment Bureau has called the EU’s ambitious goals of self-reliance and the increase of intraregional production of critical materials and batteries a double-edged sword, arguing that environmental and societal costs must be properly assessed (Anastasio, 2020). Moreover, there have been growing concerns among environmentalists over a mining boom in the EU region since the growing relative scarcity and volatile global political conditions has led the EU to seek a more secure supply of materials from Europe. So, the outsourced supply chains of materials from mining are now thought of to be better produced inside the region. In order to make these plans work, the EC hopes to increase public acceptance of mining with the argument that resource extraction is necessary to meet climate goals and offering a narrative where mining is associated with sustainability. (Marin, 2020).
But how could an industry like mining even be marketed as something sustainable when the environmental and social impacts of the industry are known to be an issue? Lithium mining for example may have potential environmental impacts both in extraction and in processing. Concerns are related to air, water and soil pollution as well as the depletion of water resources. Research has also shown that environmental impact evaluation tools, such as life-cycle assessment, are limited in mining because of a lack of properly defined quantifiable impact categories and functional units. On a more positive note however, evidence does also suggest that lithium mining methods can be improved in a way that protects social and environmental systems without compromising economics and that alternative technologies also offer alternative ways to improve lithium extraction and processing (Kaunda, 2020, 241-243). Moreover, there are also concerns over the promotion of mining activities inside the EU because of possible social inequalities within Europe that mining projects entail.
Mining projects have the potential of putting the lives of people and wildlife at risk since mines are often set up in areas near mountains and rivers. An example of such a scenario is an EU-backed lithium mine in Caceres, Spain, Infinity Lithium’s San José de Valdeflórez lithium project, where the mine would be located a mere 800 meters from the town’s historic center which is a World Heritage site and an important location for tourism. Locals have voiced their concerns over their right of self-determination and fears of not being heard in the process of developing such mining activities (Marin, 2020, Macintosh, 2018). Environmental justice is also something to consider. The mining activities in Caceres have been reported to lack necessary permits and have even been prohibited by Caceres own General Urban Development Plan. Also, a letter of opposition signed by 134 organizations, a letter directed at President of the European Commission Ursula von der Leyen, has also tried to highlight the worries of local communities over the mining project’s likely impacts on the environment, water as well as central local economic activities of tourism. The mining project is also understood to go against the intentions of EU’s Biodiversity and European Green Deal strategies. Concerns have also been raised over the mine’s lack of social license to operate, SLO. The EU’s goals to make new frontiers of extractive industries in Europe must also then include the tackling of issues surrounding lack of proper standards, transparency, and community consultation if community opposition are to be avoided (Rhoades, 2020). Similar stories come from a village of Covas do Barroso in Portugal. Plans to excavate lithium form the largest estimated deposits of lithium in Western Europe are against the interests of locals (Carter, 2021). Aspects related to social inequalities and environmental justice are then issues the EU needs to work on if the union wants to see just, inclusive transitions to sustainable, carbon neutral lifestyles. Calls have also been made to revise the Circular Economy Action Plan to correspond more accurately to true circular economy objectives (Friends of the Earth Europe, 2020). The cases discussed above are just a few examples of how the EU’s visions of low-carbon futures might not always be welcomed in Europe. Surely, new mining projects will be needed for the ambitious climate and sustainability goals, but if negative impacts of mining are most greatly felt in local communities, it raises a question of will new mining projects lead to more inequality in Europe with the price of a more equal market role for the EU in the critical raw materials market. In the end, is the growing mining boom really about inclusive and just futures or just a short-term technical fix in the long-term battle against climate change?
Finland and lithium
For a transition to a carbon neutral economy to happen smoothly, the supply of materials needed for technology that enables said carbon neutrality must also be smooth. In the case of lithium supply, security has become a top priority for technology companies. This new focus on secure supply has resulted in joint ventures among exploration companies and technology companies to guarantee a reliable and diverse supply of lithium for manufactures and suppliers. Finland is listed as a potential location for mineral-based lithium sources (United States Geological Survey, 2020). But will Finland face similar events related to mining activities as in other above-mentioned examples from Europe? Most likely not to the same extent. A current lithium mining project of Keliber in Kaustinen and Kokkola seems to have been received positively, and reports indicate that due to its remote location the mining projects won’t have many negative impacts on the surrounding community. Moreover, when in operation, Keliber will become an important regional employer. Environmental assessments on various factors such as groundwaters, surface waters and vegetation seem to also indicate that environmental impacts will be small or moderate (Keliber, 2020). The Keliber project has been discussed in the media to be exceptionally unproblematic in terms of location and the size of the mineral deposits. Some concerns have been raised over the possible issues of profit distribution but the general opinion about it also seems to lean towards more positive (Toivonen, 2021). However, local landowners as well as the Finnish Association for Nature Conservation have voiced their concerns about Keliber’s environmental permit procedures and the possible negative impacts of the mining project to the local environment and communities (Slotte, 2019, Vihanta, 2018). But overall, in terms of lithium mining, Finland may fare rather well without major negative impacts. The Keliber mining project seems to be positively received and has gone through a strict environmental impact assessment. However, negative spillovers concerning lithium can’t be discussed further because the Keliber mining project isn’t in operation yet, but the process up till now seems to lean towards having more positive than negative impacts in the Finnish context.
Anastasio, M. (2020). Europe’s strategy for critical raw materials “a double-edged sword”. https://eeb.org/europes-strategy-for-critical-raw-materials-a-double-edged-sword/ . Accessed: 23.4.2021.
Bobba, S., Carrara, S., Huisman, J., Mathieux, F., Pavel, C. (2020). Critical Raw Materials for Strategic Technologies and Sectors in the EU: A Foresight Study. https://rmis.jrc.ec.europa.eu/uploads/CRMs_for_Strategic_Technologies_and_Sectors_in_the_EU_2020.pdf . Accessed: 23.4.2021.
Carter, B. (2021). A Portuguese village pays the high price of low carbon energy. https://www.euronews.com/2021/04/23/portuguese-village-suffers-the-high-cost-of-low-carbon-energy . Accessed: 29.4.2021.
de Blas, I., Mediavilla, M., Capellán-Pérez, I., & Duce, C. (2020). The limits of transport decarbonization under the current growth paradigm. Energy Strategy Reviews, 32 , 100543. doi:https://doi.org/10.1016/j.esr.2020.100543
European Commission1. (2010). Europe 2020. A European strategy for smart, sustainable and inclusive growth. https://ec.europa.eu/eu2020/pdf/COMPLET%20EN%20BARROSO%20%20%20007%20-%20Europe%202020%20-%20EN%20version.pdf . Accessed: 23.4.2021.
European Commission2. (2019). COMMUNICATION FROM THE COMMISSION TO THE EUROPEAN PARLIAMENT, THE EUROPEAN COUNCIL, THE COUNCIL, THE EUROPEAN ECONOMIC AND SOCIAL COMMITTEE AND THE COMMITTEE OF THE REGIONS. The European Green Deal.
https://eur-lex.europa.eu/legal-content/EN/TXT/?qid=1596443911913&uri=CELEX:52019DC0640#document2. Accessed: 29.4.2021.
European Commission3. (2020). Green Deal: Sustainable batteries for a circular and climate neutral economy.
https://ec.europa.eu/commission/presscorner/detail/en/ip_20_2312. Accessed: 29.4.2021.
European Commission4. (2020). Questions and Answers on Sustainable Batteries Regulation.
https://ec.europa.eu/commission/presscorner/detail/en/qanda_20_2311 . Accessed: 23.4.2021.
European Commission5. (2020). COMMUNICATION FROM THE COMMISSION TO THE EUROPEAN PARLIAMENT, THE COUNCIL, THE EUROPEAN ECONOMIC AND SOCIAL COMMITTEE AND THE COMMITTEE OF THE REGIONS Critical Raw Materials Resilience: Charting a Path towards greater Security and Sustainability.
https://eur-lex.europa.eu/legal-content/EN/TXT/?uri=CELEX:52020DC0474 . Accessed: 27.4.2021.
European Commision6. Internal Market, Industry, Entrepreneurship and SMEs. Critical Raw Materials.
https://ec.europa.eu/growth/sectors/raw-materials/specific-interest/critical_en . Accessed: 27.4.2021.
Friends of the Earth Europe. (2020). A circular economy within ecological limits.
https://friendsoftheearth.eu/publication/a-circular-economy-within-ecological-limits/ . Accessed: 29.4.2021.
Kaunda, R. B. (2020). Potential environmental impacts of lithium mining. Journal of Energy & Natural Resources Law, 38 (3), 237-244. doi:10.1080/02646811.2020.1754596
Kavanagh, L., Keohane, J., Garcia Cabellos, G., Lloyd, A., & Cleary, J. (2018). Global lithium Sources—Industrial use and future in the electric vehicle industry: A review. Resources (Basel), 7 (3), 57. doi:10.3390/resources7030057
Keliber. (2020). Keski-Pohjanmaan litiumprovinssin laajennuksen YVA-selostus. https://www.keliber.fi/site/assets/files/2386/yva_selostus_keliber_2020_24112020.pdf . Accessed: 29.4.2021.
Macintosh, E. (2018). Thousands take to streets to protest Spanish mining boom. https://meta.eeb.org/2018/02/13/public-outcry-over-spanish-mining-boom/ . Accessed: 27.4.2021.
Marin, D. F. (2020). Europe’s scramble for minerals comes home. https://meta.eeb.org/2020/09/10/europes-raw-materials-ambitions-and-the-right-to-say-no/ . Accessed: 24.4.2021.
OECD global material resources outlook to 2060: Economic drivers and environmental consequences (2019). OECD.
Rhoades, H. (2020). Cáceres rejects lithium mine. https://theecologist.org/2020/jun/10/caceres-rejects-lithium-mine . Accessed: 29.4.2021.
Slotte, C-O. (2019). Keliberin ensimmäinen louhos sai ympäristöluvan, mutta rahoitus on edelleen auki – SLL aikoo jättää valituksen. https://www.keskipohjanmaa.fi/uutinen/570595 . Accessed: 29.4.2021.
Toivonen, J. (2021). Analyysi: Kaustisen suurta litiumkaivosta on vaikea vastustaa, niin hyvältä kaikki näyttää – hankkeessa on vain yksi kipukohta. https://yle.fi/uutiset/3-11805712. Accessed: 29.4.2021.
United States Geological Survey. (2020). Lithium. Mineral Commodity Summaries . https://pubs.usgs.gov/periodicals/mcs2020/mcs2020-lithium.pdf . Accessed: 27.4.2021.
Vihanta, A. (2018). Maanomistajat kritisoivat Keliberin litiumkaivoksen vesien käsittelyä – näkevät lupahakemuksessa paljon puutteita.
https://yle.fi/uutiset/3-10413889 . Accessed: 29.4.2021.
Mining of nickel: Negative Environmental Impacts
Text by eemi saarinen.
Why is nickel so crucial for green technology?
When it comes to green technology, there is often a lot of talk about lithium-ion batteries, which serve as a power source to electric cars, for example. However, despite its name, lithium-ion batteries are actually mostly produced with nickel, alongside cobalt and lithium. This has been the case so far, but there are also some actions against the use of nickel. In February 2021 the owner of Tesla, Elon Musk, announced that Tesla will in some cars replace nickel with iron (Li, 2021). Previously he has pleaded for greater production of nickel. Despite the decision of the electric car giant’s decision, the need of nickel in creating green technology is still massive. How nickel is produced globally and in Finland, and what might be the negative environmental spillover effects? How sustainable is nickel production when examining it with the SDGs of the UN?
Nickel globally and in Finland
Globally the mining production of nickel was 2,7 million tons in 2019 (Government of Canada, 2021). The growth has been significant since 2010 (not constant, though), when production of nickel was only 1,6 million tons annually. Although we are interested in nickel as material used in batteries, it must be mentioned that batteries account only 4 % of global use of nickel (Government of Canada, 2021). 71 % of nickel is used to produce stainless steel, so the electric car boom does not account for very much of the global rise of nickel production. The sovereign leader of nickel production globally is Indonesia, accounting for 29,8 % of total production. The five next greatest producers are Philippines, Russia, New Caledonia, Canada and Australia, as can be seen in the table below:
The production of nickel in Finland has grown over the past decade. Right now, there are three major mines in Finland mining nickel: Terrafame’s mine in Talvivaara, and Boliden’s mines in Kylylahti and Kevitsa (Vasara, 2019). In 2018, the total amount of nickel mined was 43 752 tons. 212 069 tons of nickel concentrate was produced. In addition, Boliden has a nickel smelter in Harjavalta, which uses nickel concentrates from the Kevitsa mine (Boliden, 2020). The smelter’s importance is significant, because it is the only nickel smelter in Western Europe. Nornickel also produces nickel products in Harjavalta.
Negative environmental impacts of nickel and the SDGs
Mining and smelting of nickel may have many environmental impacts. In Finland, there are some examples of environmental problems caused by nickel. In 2014, Nornickel’s factory leaked 66 tons of nickel sulfate in Kokemäki river, causing the death of millions of endangered freshwater pearl mussels (Centre for Economic Development, Transport and the Environment, 2017). The leak led to weakening of the entire river ecosystem, because mussels filter for example plankton and alga from the water. Concentration of nickel in the water exceeded the environmental quality norm four-hundredfold, therefore the overall quality of water was affected enormously. This example makes it clear that mining activities needed to create “green technology” can be all but green. Of course, this example illustrates a case of a single catastrophic event, but it is important to assess negative environmental spillover effects produced by everyday activity.

Even when nothing disastrous happens, there are some factors that can make one question, if mining nickel for green technology is so green after all. Nickel must be extracted from the ore with smelting, which requires a high amount of energy and produces emissions (Dunn, Gaines, Kelly, James & Gallagher, 2014). Sulfur oxide emissions are usually connected to smelting of nickel. For example in Canada sulfur dioxide emissions have caused some serious environmental damage: acid rain emissions, heavy metal soil contamination, wetland acidification and biodiversity loss, to name a few (Dunn et al., 2014). When it comes to the high energy intensity of smelting, it is important to consider whether the energy used is renewable? Because if not, creating “green technology” using high amounts of nonrenewable energy might be just another form of greenwashing. Emissions and amount of energy used relate also to laterite ore, from which nickel is often extracted. Mudd (2010) points out sustainability problems of the nickel industry: there is an evident decline in long-term ore rates in the nickel industry, meaning that more and more laterite must be processed to extract the desired amount of nickel. This is problematic in terms of energy consumption, especially when the energy used is not renewable.
There are a few main environmental issues of nickel production, which are in contrast with the UN’s SDGs. Violating the SDGs can be challenging to observe. There are some concrete targets set by the UN, alongside global indicators. However, they might not be sufficiently specific to address mining/local issues. Nevertheless, it is probable that nickel producing might be in contrast with the following environmental SDGs:
6: Clean water and sanitation, 7: Affordable and clean energy, 13: Climate action, 14: Life below water and 15: Life on land
It is evident that production of nickel may pollute water and damage life below water. The mussel deaths in Finland, and damage to soil and wildlife both below water and on land in Canada show that green technology does not come without risks for the environment. One could also question the positive effect on creating affordable and green energy and climate action, if nickel is not mined and refined using renewable energy. At least we must be aware of the possible negative environmental impacts of nickel, when we are creating so called green technology.
Boliden (2020) Boliden harjavalta. Retrieved from https://www.boliden.com/operations/smelters/boliden-harjavalta
Centre for Economic Development, Transport and the Environment. (2017, June 21). Kokemäenjoen kesän 2014 nikkelipäästö aiheutti korjattavaksi määrätyn merkittävän vesistö- ja luontovahingon (Varsinais-Suomi ja Satakunta). Retrieved from https://www.ely-keskus.fi/-/kokemaenjoen-kesan-2014-nikkelipaasto-aiheutti-korjattavaksi-maaratyn-merkittavan-vesisto-ja-luontovahingon-varsinais-suomi-ja-satakunta-#.WUpAd2YUmUk
Dunn, J. B., Gaines, L., Kelly, J. C., James, C., & Gallagher, K. G. (2015). The significance of Li-ion batteries in electric vehicle life-cycle energy and emissions and recycling’s role in its reduction. Energy & Environmental Science , 8 (1), 158-168. https://doi.org: 10.1039/C4EE03029J
Government of Canada (2021, February 22). Nickel facts. Retrieved from https://www.nrcan.gc.ca/our-natural-resources/minerals-mining/minerals-metals-facts/nickel-facts/20519
Mudd, G. M. (2010). Global trends and environmental issues in nickel mining: Sulfides versus laterites. Ore Geology Reviews, 38(1-2), 9-26. https://doi.org/10.1016/j.oregeorev.2010.05.003
Li, Y. (2021, February 26). Musk Says Nickel Is ‘Biggest Concern’ For Electric-Car Batteries. Bloomberg. Retrieved from https://www.bloomberg.com/news/articles/2021-02-25/musk-says-nickel-is-biggest-concern-for-electric-car-batteries
Vasara, H. (2019). Toimialaraportit – Kaivoteollisuus Report prepared for Ministry of employment and the economy of Finland https://julkaisut.valtioneuvosto.fi/bitstream/handle/10024/161860/TEM_2019_57.pdf
2 Replies to “Exploring the Negative Impacts of Mining Minerals”
Hello there I am so grateful I found your blog page, I really found you by accident, while I was researching on Google for something else, Nonetheless I am here now and would just like to say cheers for a fantastic post and a all round exciting blog (I also love the theme/design), I don’t have time to read through it all at the minute but I have book-marked it and also added your RSS feeds, so when I have time I will be back to read a great deal more, Please do keep up the superb work.
Heya i am for the first time here. I came across this board and I in finding It really useful & it helped me out a lot. I am hoping to give something again and aid others such as you helped me.
Leave a Reply Cancel reply
Your email address will not be published. Required fields are marked *
Save my name, email, and website in this browser for the next time I comment.
- Help & FAQ
A lithium-isotope perspective on the evolution of carbon and silicon cycles
- Geosciences
- High Meadows Environmental Institute
Research output : Contribution to journal › Article › peer-review
The evolution of the global carbon and silicon cycles is thought to have contributed to the long-term stability of Earth’s climate 1–3 . Many questions remain, however, regarding the feedback mechanisms at play, and there are limited quantitative constraints on the sources and sinks of these elements in Earth’s surface environments 4–12 . Here we argue that the lithium-isotope record can be used to track the processes controlling the long-term carbon and silicon cycles. By analysing more than 600 shallow-water marine carbonate samples from more than 100 stratigraphic units, we construct a new carbonate-based lithium-isotope record spanning the past 3 billion years. The data suggest an increase in the carbonate lithium-isotope values over time, which we propose was driven by long-term changes in the lithium-isotopic conditions of sea water, rather than by changes in the sedimentary alterations of older samples. Using a mass-balance modelling approach, we propose that the observed trend in lithium-isotope values reflects a transition from Precambrian carbon and silicon cycles to those characteristic of the modern. We speculate that this transition was linked to a gradual shift to a biologically controlled marine silicon cycle and the evolutionary radiation of land plants 13,14 .
All Science Journal Classification (ASJC) codes
Access to document.
- 10.1038/s41586-021-03612-1
Other files and links
- Link to publication in Scopus
- Link to the citations in Scopus
Fingerprint
- Carbon Cycle Medicine & Life Sciences 100%
- Silicon Medicine & Life Sciences 79%
- Lithium Medicine & Life Sciences 71%
- Isotopes Medicine & Life Sciences 71%
- Lithium Carbonate Medicine & Life Sciences 47%
- Carbonates Medicine & Life Sciences 23%
- Seawater Medicine & Life Sciences 21%
- Radiation Medicine & Life Sciences 12%
T1 - A lithium-isotope perspective on the evolution of carbon and silicon cycles
AU - Kalderon-Asael, Boriana
AU - Katchinoff, Joachim A.R.
AU - Planavsky, Noah J.
AU - Hood, Ashleigh v.S.
AU - Dellinger, Mathieu
AU - Bellefroid, Eric J.
AU - Jones, David S.
AU - Hofmann, Axel
AU - Ossa, Frantz Ossa
AU - Macdonald, Francis A.
AU - Wang, Chunjiang
AU - Isson, Terry T.
AU - Murphy, Jack G.
AU - Higgins, John A.
AU - West, A. Joshua
AU - Wallace, Malcolm W.
AU - Asael, Dan
AU - Pogge von Strandmann, Philip A.E.
N1 - Publisher Copyright: © 2021, The Author(s), under exclusive licence to Springer Nature Limited.
PY - 2021/7/15
Y1 - 2021/7/15
N2 - The evolution of the global carbon and silicon cycles is thought to have contributed to the long-term stability of Earth’s climate1–3. Many questions remain, however, regarding the feedback mechanisms at play, and there are limited quantitative constraints on the sources and sinks of these elements in Earth’s surface environments4–12. Here we argue that the lithium-isotope record can be used to track the processes controlling the long-term carbon and silicon cycles. By analysing more than 600 shallow-water marine carbonate samples from more than 100 stratigraphic units, we construct a new carbonate-based lithium-isotope record spanning the past 3 billion years. The data suggest an increase in the carbonate lithium-isotope values over time, which we propose was driven by long-term changes in the lithium-isotopic conditions of sea water, rather than by changes in the sedimentary alterations of older samples. Using a mass-balance modelling approach, we propose that the observed trend in lithium-isotope values reflects a transition from Precambrian carbon and silicon cycles to those characteristic of the modern. We speculate that this transition was linked to a gradual shift to a biologically controlled marine silicon cycle and the evolutionary radiation of land plants13,14.
AB - The evolution of the global carbon and silicon cycles is thought to have contributed to the long-term stability of Earth’s climate1–3. Many questions remain, however, regarding the feedback mechanisms at play, and there are limited quantitative constraints on the sources and sinks of these elements in Earth’s surface environments4–12. Here we argue that the lithium-isotope record can be used to track the processes controlling the long-term carbon and silicon cycles. By analysing more than 600 shallow-water marine carbonate samples from more than 100 stratigraphic units, we construct a new carbonate-based lithium-isotope record spanning the past 3 billion years. The data suggest an increase in the carbonate lithium-isotope values over time, which we propose was driven by long-term changes in the lithium-isotopic conditions of sea water, rather than by changes in the sedimentary alterations of older samples. Using a mass-balance modelling approach, we propose that the observed trend in lithium-isotope values reflects a transition from Precambrian carbon and silicon cycles to those characteristic of the modern. We speculate that this transition was linked to a gradual shift to a biologically controlled marine silicon cycle and the evolutionary radiation of land plants13,14.
UR - http://www.scopus.com/inward/record.url?scp=85111114312&partnerID=8YFLogxK
UR - http://www.scopus.com/inward/citedby.url?scp=85111114312&partnerID=8YFLogxK
U2 - 10.1038/s41586-021-03612-1
DO - 10.1038/s41586-021-03612-1
M3 - Article
C2 - 34262211
AN - SCOPUS:85111114312
SN - 0028-0836
JO - Nature
JF - Nature
Tracing Si–N–P ecosystem-pathways: is relative uptake in riparian vegetation influenced by soil waterlogging, mowing management and species diversity?
- Wetland Restoration
- Open access
- Published: 18 May 2011
- Volume 674 , pages 41–50, ( 2011 )
Cite this article
You have full access to this open access article
- Eric Struyf 1 ,
- Wiktor Kotowski 2 ,
- Sander Jacobs 1 ,
- Stefan Van Damme 1 ,
- Kris Bal 1 ,
- Wout Opdekamp 1 ,
- Hans Backx 1 ,
- Dimitri Van Pelt 1 &
- Patrick Meire 1
1727 Accesses
6 Citations
Explore all metrics
Despite the growing concern about the importance of silicon (Si) in controlling ecological processes in aquatic ecosystems, little is known about its processing in riparian vegetation, especially compared to nitrogen (N) and phosphorus (P). We present experimental evidence that relative plant uptake of N and P compared to Si in riparian vegetation is dependent on mowing practices, water-logging and species composition. Results are obtained from a controlled and replicated mesocosm experiment, with a full-factorial design of soil water logging and mowing management. In our experiments, the Si excluding species Plantago lanceolata was dominant in the mown and non-waterlogged treatments, while Si accumulating meadow grasses and Phalaris arundinacea dominated the waterlogged treatments. Although species composition, management and soil moisture interacted strongly in their effect on relative Si:N and Si:P uptake ratios, the uptake of N to P remained virtually unchanged over the different treatments. Our study sheds new light on the impact of riparian wetland ecosystems on nutrient transport to rivers. It indicates that it is essential to include Si in future studies of the impact of riparian vegetation on nutrient transport, as these are often implemented as a measure to moderate excessive N and P inputs.
Similar content being viewed by others
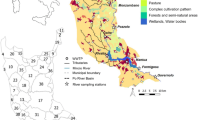
Soil system budgets of N, Si and P in an agricultural irrigated watershed: surplus, differential export and underlying mechanisms
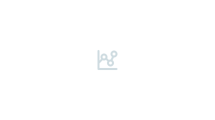
Silicon Affects Nutrient Content and Ratios of Wetland Plants
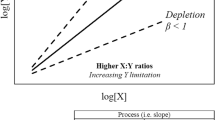
Evaluation of nutrient stoichiometric relationships among ecosystem compartments of a subtropical treatment wetland. Do we have “Redfield wetlands”?
Avoid common mistakes on your manuscript.
Introduction
The uptake, storage and recycling of silicon (Si) in ecosystem biomass and soils are now recognized as important components of silicon transport through the terrestrial environment (Conley, 2002 ; Derry et al., 2005 ; Street-Perrott & Barker, 2008 ; Struyf & Conley, 2009 ), but the relative importance of the biological buffer in different ecosystems, and the impact of environmental forcing factors on the efficiency of storage, uptake and recycling still remains poorly quantified. Si is a key nutrient in determining the species composition of aquatic and coastal phytoplankton communities (Cloern, 2001 ). The commonly observed effect of increased anthropogenic inputs of nitrogen (N) and phosphorus (P) to aquatic ecosystems is a shift in the coastal phytoplankton community to one dominated by dinoflagellates (Lotze et al., 2006 ), caused by dissolved Si (DSi) limitation of diatoms (Conley et al., 1993 ). A lack of DSi can lead to a less efficient food web structure as well as to hypoxia and harmful blooms of toxic algae (e.g. Anderson et al., 2002 ). Silicon also plays an essential role in the global carbon (C) cycle. A continuous import of Si from the terrestrial environment is necessary to maintain the biological C pump in the ocean (Tréguer & Pondaven, 2000 ), and mineral weathering of silicate minerals is one of the major terrestrial sinks for carbon dioxide (CO 2 ) (Berner, 1992 ). The poor quantification of the biological Si buffer in ecosystems forms a prime challenge in constraining the silicon cycle related C sinks.
Wetlands and meadows are prime candidates to form a hot-spot in the biological Si buffer, as they can accumulate large amounts of biogenic amorphous Si (ASi) (Blecker et al., 2006 ; Struyf & Conley, 2009 ) in the form of plant phytoliths, diatoms and sponge spicules. Riparian meadows are well-established buffers in the N and P cycle. They affect the output of these nutrients to rivers through permanent or temporary uptake in vegetation, detritus and soils and through transformation processes, e.g. removal by denitrification (e.g. Güsewell, 2005 ; Olde Venterink et al., 2006 ). Riparian wetlands are often (re)established as a countermeasure against excessive N and P export from agricultural catchments. Up to now however, little research has focused on the influence of ecosystem characteristics on Si processing in riparian meadows or wetlands. Management has been observed to potentially interfere with the storage of ASi near the surface of riparian wetlands (Struyf et al., 2009 ), but experimental evidence is currently lacking (Struyf & Conley, 2009 ).
We analyzed uptake of Si by riparian vegetation in reference to N and P in mesocosm communities grown for one season under contrasting moisture and management conditions. Earlier experiments have focused on N, P and often K and C, while failing to include Si. Our study sheds new light on the impact of riparian wetland ecosystems on nutrient transport to rivers, and is new evidence of the importance of the role of vegetation in terrestrial Si fluxes.
Materials and methods
We established a full-factorial mesocosm experiment with soil water-logging and summer mowing as factors, each of them in two levels (waterlogged, anoxic vs. non-waterlogged, oxic and mown vs. unmown). The experimental set-up is described in Kotowski et al. ( 2010 ). Briefly, mesocosms were composed of PVC containers of 91 × 111 cm surface and 61 cm height. Outflow taps located at the bottom of side walls connected to PVC pipes allowed for the control of the water level. Containers were filled with 20 cm of drainage substrate (Argex clay aggregate, Argex NV, Zwijndrecht, Belgium) separated by geo-textile from a 30 cm cover of a soil mixture containing 3:1 volumetric parts of fen peat and alluvial clay. Mixing fen peat and clay ensured that the soil would have high water potential and mineral content (clay) and at the same time, high organic matter content (peat) necessary to produce reduced conditions after water-logging. Presence of peat in the soil also allowed for easier percolation of water under non-water logged conditions. To maintain eutrophic conditions throughout the experiment, an initial amount of 168 g N, 192 g P, 216 g potassium (K) and 24 g magnesium (Mg) per m 3 soil in an easily soluble form (PG-mix Floranid, COMPO Benelux nv, Deinze, Belgium), and a slow release fertilizer (6 months release time; Osmocote, SCOTTS Professional, Sint-Niklaas, Belgium) containing 300 g N, 180 g P and 180 g K per m 3 soil were added. These values are at the upper limit of annual nutrient input in European floodplains (Olde Venterink et al., 2006 ).
Forty mesocosms were placed in a block of 4 × 10. Water was supplied by sprinklers installed 30 cm above each container once (spring, autumn) or twice (summer) a day. Watering time depended on the time of the year (between 5 and 25 min per day) and specifically ensured that plants were not limited by water shortage. Excessive water could flow out of the system through the outflow pipe. In anoxic soils, the water level was held constant at 2–5 cm below soil surface, while the outflow was levelled at the bottom of the containers in the oxic soils.
The species used in the experiment were typical of Central European floodplains. We carefully selected species as to have a similar representation of typical fen, wet meadow and mesic meadow species and to assure that each of these groups contained species from various functional types and growth forms (Supplementary material). A seed mixture of 37 species was introduced on the 15th of May 2006 in a density of approximately 1,000 seeds m −2 species −1 . Soil moisture measurements at 5 cm depth carried out in mid June between two watering treatments confirmed differences between the waterlogged and the non-waterlogged treatments (soil moisture: 54.2% ± 12.1 and 32.4% ± 3.5, respectively, by weight, t test P < 0.001).
The above-ground biomass of the 20 mesocosms subjected to the summer mowing treatment was harvested at the end of June 2006. Biomass was sorted to species level, except for the meadow grasses which were grouped. Dry biomass of all species was determined separately (except for the meadow grasses) in each mesocosm after 72 h of drying at 70°C. At the end of the experiment (September 2006), the above-ground biomass of all 40 mesocosms was harvested and the sorting and drying was carried out as described above.
Nutrient analysis
N, P and Si concentrations in plant biomass for all established species were determined. If a species established in more than seven mesocosms with the same combination of mowing and aeration treatment, seven randomly chosen replicates were analyzed for their N, P and Si content.
After drying, the plant material was grounded with a motor mill and analyzed for N, P and Si content. ASi content was analyzed through incubation of 25 mg of ground, homogenized plant material for 4 h in 0.1 M Na 2 CO 3 at 80°C (DeMaster, 1981 ). Extracted dissolved silicon was subsequently analyzed spectrophotometrically on a Thermo IRIS ICP (Inductively Coupled Plasmaspectrophotometer). N and P content were analyzed according to Walinga et al. ( 1989 ) on a colorimetric segmented flow analyzer.
Nutrient uptake at the vegetation level
To allow the analysis of nutrient uptake at community level during the experiment (May–September 2006), the dry weight of every species was multiplied with the average N, P and Si content observed for the species in a certain treatment. The total N, P and Si uptake in biomass for every mesocosm was then calculated by summation of the calculated total nutrient uptake in all species. For the mown treatments, this is the summation of the May–June and July–September uptake.
Statistical analyses
A one-way ANOVA analysis was used to comparing the treatments for different variables (Biomass, N, P and Si uptake, N:P ratio, N:Si and P:Si). Classification of the treatments was performed with Post-hoc Scheffe tests. All analyses were performed in SPSS (Statistical Package for the Social Sciences) 14.0 (SPSS, 2005 ).
Vegetation patterns and biomass
In total, 26 species established in the mesocosms but only 14 were present in sufficient amounts to allow for chemical analyses (see Kotowski et al., 2010 for detailed information on species recruitment in each treatment). Three meadow grasses established (i.e. Lolium perenne L. , Poa pratensis L., and Alopecurus pratensis L.): these were grouped as one taxon within the analysis. Another grass species, Phalaris arundinacea L. was considered separately, as it is a typical wetland grass, while the other grouped species are typical meadow grasses. As such, 11 species and one group-taxon were included in the analysis: “meadow grasses ( L. perenne , P. pratensis and A. pratensis )”, Achillea millefolium L., Centaurea jacea L., Cirsium oleraceum L. Scop., Daucus carota L., Epilobium hirsutum L., Leontodon autumnalis L., Lycopus europaeus L., Lythrum salicaria L., P. arundinacea , Plantago lanceolata L. and Valeriana officinalis L..
The total above-ground biomass was higher in the non-waterlogged, oxic treatments (Table 1 ). Biomass in oxic treatments was dominated by P. lanceolata , and to a lesser extent, by P. arundinacea , D. carota and A. millefolium (Fig. 1 ). Biomass in the waterlogged, anoxic treatments was dominated by meadow grasses and P. lanceolata , and to a lesser extent by P. arundinacea (Fig. 1 ). A clear shift of the dominant species was apparent between anoxic and oxic treatments, with meadow grasses dominating the anoxic treatments and P. lanceolata the oxic treatments (Fig. 1 ). More than 80% of the decrease in total biomass under anoxic conditions could be attributed to P. lanceolata , with a strong decline in biomass, both in mown (−1,000 g dry weight m −2 of P. lanceolata biomass on average) and unmown mesocosms (−1,360 g dry weight m −2 on average). Given the importance of P. lanceolata for total biomass production, we also tested for differences in biomass excluding P. lanceolata . Although a marginally significant effect of treatment on biomass without P. lanceolata biomass existed ( P < 0.04), the post-hoc Scheffe tests did not separate any of the treatments individually (Table 1 ).
Contribution of different species to total biomass in the different treatments (averaged, n = 10 for every treatment)
Nutrient concentrations at the species level
The plant nutrient concentration among the different replicates only showed small variability (Table 2 ). Results for nutrient contents will focus on the main dominant species D. carota , A. millefolium , “meadow grasses”, P. arundinacea and P. lanceolata , which represented over 90% of nutrient uptake. Average biomass for the dominant species, and average N, P and Si concentrations in the plant tissue is summarized in Table 2 . P. lanceolata and D. carota had low N, P and Si contents compared to the other dominant species . The species in the anoxic treatment generally contained less N, P and Si compared to the oxic treatments. Highest relative differences were observed for Si content in P. arundinacea , meadow grasses and A. millefolium , which had more than twice the relative Si content in oxic compared to anoxic treatments.
Nutrient uptake
Total uptake of nutrients (N, P and Si) in the oxic treatments was high compared to anoxic treatments (Fig. 2 ; Table 1 ). Under oxic circumstances, N and P uptake were higher in the mown treatments; this effect was not apparent for Si uptake (Table 1 ). The differences for N and P uptake were attributable to P. lanceolata biomass. Excluding this, no differences in total N and P uptake were apparent (Table 1 , Fig. 2 lower panel). This was not the case for Si uptake; excluding P. lanceolata biomass had no effect on Si uptake (Table 1 ; Fig. 2 ) as there is almost no Si in P. lanceolata (Table 2 ).
Total nitrogen (N), phosphorus (P) and silicon (Si) uptake in the vegetation of the different treatments. The top panels are for the total biomass, the lower panels for the biomass without P. lanceolata . The error bars indicate the standard deviation ( OM oxic, mown; ONM oxic, unmown; ANM anoxic, mown; ANNM anoxic, unmown)
In mown treatments more N and P were taken up compared to Si than in unmown treatments, both under oxic and anoxic conditions (Fig. 3 ; Table 1 ). The relative uptake of Si dropped mainly as a result of increasing P. lanceolata abundance in the mown treatments (N:Si ratio in P. lanceolata ranged between 17 and 38, while, e.g. P. arundinacea N:Si ranged between 2 and 5). Although significant differences existed in N:P ratio across the treatments (Table 1 ), these differences were very small (Fig. 3 ).
Nitrogen (N):phosphorus (P), P:silicon (Si), and N:Si weight ratios in the vegetation in the different treatments. The left panel is for the total biomass, the right panel for the biomass without P. lanceolata . The error bars indicate the standard deviation ( OM oxic, mown; ONM oxic, unmown; ANM anoxic, mown; ANNM anoxic, unmown)
If we remove P. lanceolata from the analysis, all remaining dominant vegetation consists of Si accumulators. This had little effect on N:P ratio in the vegetation across the treatments (Fig. 3 ; Table 2 ), although small significant differences still existed (Table 2 ). Removing P. lanceolata from the analysis had almost no effect on the total Si content of plants, while the N and P taken up by the vegetation decreased drastically (Fig. 2 ). The N:Si and P:Si ratio in the Si accumulator (non- Plantago ) biomass was mainly related to the water-logging in the soil (Table 1 , Fig. 3 ), although mowing had a significant effect in the anoxic treatments (Table 1 ).
In summary, the N:P ratio only differed marginally over species composition, soil moisture and mowing conditions. In contrast, the ratio at which Si was taken up relative to the other nutrients was significantly different. Mowing enhanced occurrence of P. lanceolata , and as such decreased relative uptake of Si. In the accumulator biomass, relative uptake of Si to N and P was mainly related to water logging.
Although abundant experimental studies have focused on N and P uptake in riparian vegetation, a controlled experiment which studies Si uptake (relative to N and P) in detail, under changing environmental conditions, is surprisingly lacking. Riparian meadows, of which our experimental units were small-scale versions, have a high potential to influence the Si input into rivers. Often dominated by Si-rich grasses or sedges, they are likely among the most active processors of Si of all terrestrial ecosystems (e.g. Blecker et al., 2006 ; Sommer et al., 2006 ; Struyf & Conley, 2009 ).
P concentrations in our experiment (the 4 dominant species contain on average 3.7 mg g −1 dry weight) were high compared to other studies, while N concentrations (18.7 mg g −1 dry weight) were high but not uncommon (Güsewell & Koerselman, 2002 ). Our experiment was, given the high supply of N and P through fertilisers and the relatively high concentrations of N and P in plant tissues, clearly representative for a set of riparian meadows in an eutrophied environment, with N less available compared to P (but neither should be considered limiting at measured concentrations). In such eutrophied environments, wetland restoration and protection is often implemented as a measure to reduce N and P export. It is a well-established concept that controlling N and P export upstream by implementing riparian vegetations is one of the most effective ways to control N and P fluxes through river basins, and in the end, to the coastal zone (e.g. Withers & Jarvis, 1998 ; Hattermann et al., 2006 ).
Waterlogging, mowing and relative Si uptake
In our experiment, two dominant species ( P. arundinacea and A. millefolium , which contain over 1 wt% ASi) and the meadow grass cluster can be identified as Si accumulators. This is consistent with previous literature studies (Hodson et al., 2005 ). In general, monocots (e.g. Poaceae ) contain more Si than dicots. The dicot-monocot division is only a general pattern, and deviations are not an exception. Although a dicot, A. millefolium was previously identified as a substantial accumulator of silicon (Hodson et al., 2005 ). Interestingly, nearly all the species in the waterlogged treatments had a lower ASi content compared to the non-waterlogged treatments. This contrasts with the Si content in soil solution, displaying higher concentrations in waterlogged anoxic (115 ± 32 μmol l −1 ) than in oxic conditions (45 ± 5 μmol l −1 ). Dissolved Si content in soil solution and plant Si content were thus not directly linked in our experiment, although such a link was found in other experiments (e.g. Henriet et al., 2006 ).
In the experiment, relative uptake of Si in vegetation halved because of mowing practices only. This was mainly because mowing favoured the abundance of the non-Si accumulating species Plantago lanceolata . Si uptake in Si accumulators on the other hand was apparently higher under oxic conditions. This has consequences which might be important to ecosystem engineers, trying to mediate nutrient fluxes. Riparian meadows, established to reduce nutrient fluxes to the coastal zone, might remove significantly less Si, especially relative to N and P, if mowing is applied in spring and if this enhances Si-excluder growth as it did in our experiment. This could be considered a positive effect from a management point of view. Indeed, decreased relative uptake of Si compared to N and P might positively affect N–P–Si ratios in rivers and estuaries during times with risk of DSi depletion.
On the other hand, uptake of Si into grass and/or sedges has been linked to an increased burial of ASi near the surface of floodplain wetlands (Struyf et al., 2009 ). This increased storage of ASi near the surface can enrich wetland pore-waters with ASi (Struyf et al., 2005 , 2009 ), which can in turn affect the exchange of DSi between wetlands and rivers. The storage of ASi in sediment and litter has been linked to an increased DSi export during low ambient DSi concentrations in tidal rivers (Struyf et al., 2006 , 2007 ). Release of DSi from plant phytoliths has also been shown experimentally (e.g. Fraysse et al., 2010 ). Reducing the DSi buffering capacity of riparian zones through the yearly removal of the standing crop could potentially lower this Si-buffering capacity of riparian zone to the adjacent rivers. This is an undesired effect in the context of eutrophication.
Perspectives
Our understanding of Si biogeochemistry in riparian meadows, also in those constructed specifically to control nutrient fluxes, is as of yet too limited to evaluate the trade-off between the removal of N and P (and thus also Si) on the one hand, and the creation of potentially Si buffering litter layers (possibly also releasing N and P) on the other hand. In order to implement the control of Si fluxes in the design and management of riparian meadows and small-scale wetlands aimed at reduction of the local output of pollutants and N (e.g. individual factories and households, not directly connected to a general sewer and treatment system), we will need significant progress in our understanding of the functioning of these systems in the Si cycle. Both in situ and ex situ experiments, aimed at testing the importance of specific processes (such as ours) and in situ budget studies, would be valuable in this context.
Interesting topics include:
How quick is the recycling of biogenic Si in wetland and meadow soils, and how efficient is this process? Is this recycling complete, or is part of the biogenic Si buried permanently?
How much dissolved Si is exchanged between riparian meadows and adjacent rivers, and how tight is the coupling between vegetation and soil biogenic Si?
What is the in situ effect of the specific management of soil redox status, vegetation removal, drainage and hydrology?
Is it possible to optimize the nutrient ratios released from riparian meadows designed for nutrient removal, by stimulating dissolved Si release to the river, while improving N and P removal?
In the latter context, it is interesting to note that in our experiment, the Si accumulators dominated the waterlogged treatments, resulting in a higher relative Si uptake in vegetation. This will increase the reactive biogenic Si content of the riparian meadow soil, potentially resulting in an increased buffering capacity of Si available in rivers. At the same time, waterlogged conditions might favour nitrogen removal through denitrification (Hill, 1996 ), but could also favour the release of dissolved orthophosphate into pore-water and thus potentially to the river (Loeb et al., 2008 ).
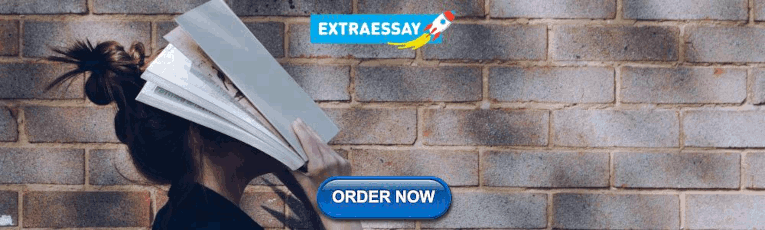
Conclusions
Understanding ecosystem resilience and buffer capacity, and how human interference has affected it, is essential to manage negative effects of human impact on these ecosystems (Gunderson, 2000 ). Land use and vegetation cover have undergone and still undergo huge changes through human intervention. For example, between 50 and 80% of wetlands have been lost from European and North-American estuaries (Elliott & Cutts, 2004 ), and have been replaced by urban or agricultural surfaces. In Midwestern States (USA), 80% of the wetland acreage has been drained (Mitsch et al., 2001 ). It has been well documented that this has reduced the resilience of aquatic systems to increased N and P concentrations, increasing the effect of excessive human N and P input. Wetland restoration programs are often based on this understanding.
In our experiments, only a small shift in vegetation coverage, induced by two changed (and manageable) environmental factors, had a relatively strong effect on the Si uptake by plants. This implies that ecosystem managers should take the potential influence of riparian meadows on Si fluxes into account, if these are restored to counteract eutrophication. However, to be able to do so, it is essential to quantify the trade-off between the two effects of Si uptake in riparian vegetation on the Si dynamics in the aquatic environment:
Temporary reduction of Si fluxes to rivers through the uptake in silicon accumulating vegetation;
The build-up of a biogenic silicon rich surface layer as a result of Si uptake into riparian vegetation, which potentially influences Si dynamics in the adjacent river.
Our concept of Si biogeochemistry in riparian meadows, also for those constructed specifically to control nutrient fluxes, is as of yet too limited to evaluate this trade-off.
Anderson, D. M., P. M. Glibert & J. M. Burkholder, 2002. Harmful algal blooms and eutrophication: nutrient sources, composition, and consequences. Estuaries 25: 704–726.
Article Google Scholar
Berner, R. A., 1992. Weathering, plants, and the long-term carbon-cycle. Geochimica et Cosmochimica Acta 56: 3225–3231.
Article CAS Google Scholar
Blecker, S. W., R. L. McCulley, O. A. Chadwick & E. F. Kelly, 2006. Biologic cycling of silica across a grassland bioclimosequence. Global Biogeochemical Cycles 20: GB3023.
Cloern, J. E., 2001. Our evolving conceptual model of the coastal eutrophication problem. Marine Ecology Progress Series 210: 223–253.
Conley, D. J., 2002. Terrestrial ecosystems and the global biogeochemical silica cycle. Global Biogeochemical Cycles 16: GB1121.
Conley, D. J., C. L. Schelske & E. F. Stoermer, 1993. Modification of the biogeochemical cycle of silica with eutrophication. Marine Ecology Progress Series 101: 179–192.
Demaster, D. J., 1981. The supply and accumulation of silica in the marine environment. Geochimica et Cosmochimica Acta 45: 1715–1732.
Derry, L. A., A. C. Kurtz, K. Ziegler & O. A. Chadwick, 2005. Biological control of terrestrial silica cycling and export fluxes to watersheds. Nature 433: 728–731.
Article PubMed CAS Google Scholar
Elliott, M. & N. D. Cutts, 2004. Marine habitats: loss and gain, mitigation and compensation. Marine Pollution Bulletin 49: 671–674.
Fraysse, F., O. S. Pokrovsky & J. D. Meunier, 2010. Experimental study of terrestrial plant litter interaction with aqueous solutions. Geochimica Et Cosmochimica Acta 74: 70–84.
Gunderson, L. H., 2000. Ecological resilience – in theory and application. Annual Review of Ecology and Systematics 31: 425–439.
Güsewell, S., 2005. High nitrogen: phosphorus ratios reduce nutrient retention and second-year growth of wetland sedges. New Phytologist 166: 537–550.
Article PubMed Google Scholar
Güsewell, S. & M. Koerselman, 2002. Variation in nitrogen and phosphorus concentrations of wetland plants. Perspectives in Plant Ecology, Evolution and Systematics 5: 37–61.
Hattermann, F. F., V. Krysanova, A. Habeck & A. Bronstert, 2006. Integrating wetlands and riparian zones in river basin modelling. Ecological Modelling 199: 379–392.
Henriet, C., X. Draye, L. Oppitz, R. Swennen & B. Delvaux, 2006. Effects distribution and uptake of silicon in banana (Musa spp.) under controlled conditions. Plant Soil 287: 359–374.
Hill, A. R., 1996. Nitrate removal in stream riparian zones. Journal of Environmental Quality 25: 743–755.
Hodson, M. J., P. J. White, A. Mead & M. R. Broadley, 2005. Phylogenetic variation in the silicon composition of plants. Annals of Botany 96: 1027–1046.
Kotowski, W., O. Beauchard, W. Opdekamp, P. Meire & R. van Diggelen, 2010. Waterlogging and canopy interact to control species recruitment in floodplains. Functional Ecology 24(4): 918–926.
Loeb, R., L. P. M. Lamers & J. G. M. Roelofs, 2008. Effects of winter versus summer flooding and subsequent desiccation on soil chemistry in a riverine hay meadow. Geoderma 145: 84–90.
Lotze, H. K., H. S. Lenihan, B. J. Bourque, R. H. Bradbury, R. G. Cooke, M. C. Kay, S. M. Kidwell, M. X. Kirby, C. K. Peterson & J. B. C. Jackson, 2006. Depletion, degradation, and recovery potential of estuaries and coastal seas. Science 312: 1806–1809.
Mitsch, W. J., J. W. Day, J. W. Gilliam, P. M. Groffman, D. L. Hey, G. W. Randall & N. Wang, 2001. Reducing nitrogen loading to the gulf of Mexico from the Mississippi river basin: strategies to counter a persistent ecological problem. Bioscience 51: 373–388.
Olde Venterink, H., J. E. Vermaat, M. Pronk, F. Wiegman, G. E. M. van der Lee, M. W. van den Hoorn, L. Higler & J. T. A. Verhoeven, 2006. Importance of sediment deposition and denitrification for nutrient retention in floodplain wetlands. Applied Vegetation Science 9: 163–174.
Sommer, M., D. Kaczorek, Y. Kuzyakov & J. Breuer, 2006. Silicon pools and fluxes in soils and landscapes – a review. Journal of Plant Nutrition and Soil Science – Zeitschrift Fur Pflanzenernahrung Und Bodenkunde 169: 582.
SPSS, 2005. SPSS version 14.0. SPSS, Chicago, Illinois, USA.
Google Scholar
Street-Perrott, A. F. & P. A. Barker, 2008. Biogenic silica: a neglected component of the coupled global continental biogeochemical cycles of carbon and silicon. Earth Surface Processes and Landforms 33: 1436–1457.
Struyf, E. & D. J. Conley, 2009. Silica: an essential nutrient in wetland biogeochemistry. Frontiers in Ecology and Environment 7: 88–94.
Struyf, E., S. Van Damme, B. Gribsholt, J. J. Middelburg & P. Meire, 2005. Biogenic silica in tidal freshwater marsh sediments and vegetation (Schelde estuary, Belgium). Marine Ecology Progress Series 303: 51–60.
Struyf, E., A. Dausse, S. Van Damme, K. Bal, B. Gribsholt, H. T. S. Boschker, J. J. Middelburg & P. Meire, 2006. Tidal marshes and biogenic silica recycling at the land-sea interface. Limnology & Oceanography 51: 838–846.
Struyf, E., S. Van Damme, B. Gribsholt, K. Bal, O. Beauchard, J. J. Middelburg & P. Meire, 2007. Phragmites australis and Si cycling in tidal wetlands. Aquatic Botany 87: 134–140.
Struyf, E., W. Opdekamp, H. Backx, S. Jacobs, D. J. Conley & P. Meire, 2009. Vegetation and proximity to the river control amorphous Si storage in a riparian wetland (Bierbza National Park, Poland). Biogeosciences 6: 623–631.
Tréguer, P. & P. Pondaven, 2000. Global change – silica control of carbon dioxide. Nature 406: 358–359.
Walinga, I., W. Van Vark, V. J. G. Houba & J. J. Van Der Lee, 1989. Plant Analysis Procedures. Soil and Plant Analysis, Part 7, pp. 13–16. Agricultural University, Wageningen, NL: 13–16.
Withers, P. J. A. & S. C. Jarvis, 1998. Mitigation options for diffuse phosphorus loss to water. Soil Use and Management 14: 186–192.
Download references
Acknowledgments
L. Clement and E. De Bruyn analyzed Si concentration in samples in the “UA, University of Antwerp, Department of Biology, Testing Laboratory for Chemical Water Quality”. Tom Vanderspiet analyzed N and P concentrations. Eric Struyf (post-doc) and Wout Opdekamp (aspirant) acknowledge personal financing by EU Marie-Curie Programme (FP6) and/or FWO (Research Foundation Flanders). Wiktor Kotowski acknowledges the Francqui Foundation for financing his research work at Antwerp University. We are also thankful to all students, who helped in the species harvesting and sorting.
Open Access
This article is distributed under the terms of the Creative Commons Attribution Noncommercial License which permits any noncommercial use, distribution, and reproduction in any medium, provided the original author(s) and source are credited.
Author information
Authors and affiliations.
Department of Biology, Ecosystem Management Research Group, University of Antwerp, Universiteitsplein 1C, B2610 Wilrijk, Antwerp, Belgium
Eric Struyf, Sander Jacobs, Stefan Van Damme, Kris Bal, Wout Opdekamp, Hans Backx, Dimitri Van Pelt & Patrick Meire
Department of Plant Ecology and Environmental Conservation, University of Warsaw, Warsaw, Poland
Wiktor Kotowski
You can also search for this author in PubMed Google Scholar
Corresponding author
Correspondence to Wiktor Kotowski .
Additional information
Guest editors: Dominik Zak, Robert McInnes, Jörg Gelbrecht / Restoration, biogeochemistry and ecological services of wetlands
Electronic supplementary material
Below is the link to the electronic supplementary material.
Supplementary material 1 (DOC 22 kb)
Rights and permissions.
Open Access This is an open access article distributed under the terms of the Creative Commons Attribution Noncommercial License ( https://creativecommons.org/licenses/by-nc/2.0 ), which permits any noncommercial use, distribution, and reproduction in any medium, provided the original author(s) and source are credited.
Reprints and permissions
About this article
Struyf, E., Kotowski, W., Jacobs, S. et al. Tracing Si–N–P ecosystem-pathways: is relative uptake in riparian vegetation influenced by soil waterlogging, mowing management and species diversity?. Hydrobiologia 674 , 41–50 (2011). https://doi.org/10.1007/s10750-011-0737-x
Download citation
Published : 18 May 2011
Issue Date : October 2011
DOI : https://doi.org/10.1007/s10750-011-0737-x
Share this article
Anyone you share the following link with will be able to read this content:
Sorry, a shareable link is not currently available for this article.
Provided by the Springer Nature SharedIt content-sharing initiative
- Riparian meadows
- Riparian wetlands
- Eutrophication
- Find a journal
- Publish with us
- Track your research
Mining and Its Impact on the Environment Essay
Introduction, effects of mining on the environment, copper mining, reference list.
Mining is an economic activity capable of supporting the developmental goals of countries and societies. It also ensures that different metals, petroleum, and coal are available to different consumers or companies. Unfortunately, this practice entails excavation or substantial interference of the natural environment. The negative impacts of mining can be recorded at the global, regional, and local levels. A proper understanding of such implications can make it possible for policymakers and corporations to implement appropriate measures. The purpose of this paper is to describe and discuss the effects of mining on the environment.
Ways Mining Impact on the Environment
Miners use different methods to extract various compounds depending on where they are found. The first common procedure is open cast, whereby people scrap away rocks and other materials on the earth’s surface to expose the targeted products. The second method is underground mining, and it allows workers to get deeper materials and deposits. Both procedures are subdivided further depending on the nature of the targeted minerals and the available resources (Minerals Council of Australia 2019). Despite their striking differences in procedures, the common denominator is that they both tend to have negative impacts on the natural environment.
Firstly, surface mining usually requires that machines and individuals clear forests and vegetation cover. This means that the integrity of the natural land will be obliterated within a short period. Permanent scars will always be left due to this kind of mining. Secondly, the affected land will be exposed to the problem of soil erosion because the topmost soil is loosened. This problem results in flooding, contamination of the following water in rivers, and sedimentation of dams. Thirdly, any form of mining is capable of causing both noise and air pollution (Minerals Council of Australia 2019). The use of heavy machines and blasts explains why this is the case.
Fourthly, other forms of mining result in increased volumes of rocks and soil that are brought to the earth’s surface. Some of them tend to be toxic and capable of polluting water and air. Fifthly, underground mines tend to result in subsidence after collapsing. This means that forests and other materials covering the earth’s surface will be affected. Sixthly, different firms of mining are known to reduce the natural water table. For example, around 500,000,000 cubic meters of water tend to be pumped out of underground mines in Germany annually (Mensah et al., 2015). This is also the same case in other countries across the globe. Seventhly, different mining activities have been observed to produce dangerous greenhouse gases that continue to trigger new problems, including climate change and global warming.
Remediating Mine Sites
The problem of mining by the fact that many people or companies will tend to abandon their sites after the existing minerals are depleted. This malpractice is usually common since it is costly to clean up such areas and minimize their negative impacts on the natural environment. The first strategy for remediating mine sites is that of reclamation. This method entails the removal of both environmental and physical hazards in the region (Motoori, McLellan & Tezuka 2018). This will then be followed by planting diverse plant species. The second approach is the installation of soil cover. When pursuing this method, participants and companies should mimic the original natural setting and consider the drainage patterns. They can also consider the possible or expected land reuse choices.
The third remediation strategy for mine sites entails the use of treatment systems. This method is essential when the identified area is contaminated with metals and acidic materials that pose significant health risks to human beings and aquatic life (Mensah et al., 2015). Those involved can consider the need to construct dams and contain such water. Finally, mining companies can implement powerful cleanup processes and reuse or restore the affected sites. The ultimate objective is to ensure that every ugly site is improved and designed in such a way that it reduces its potential implications on the natural environment. From this analysis, it is evident that the nature of the mining method, the topography of the site, and the anticipated future uses of the region can inform the most appropriate remediation approach. Additionally, the selected method should address the negative impacts on the environment and promote sustainability.
Lessening Impact
Mining is a common practice that continues to meet the demands of the current global economy. With its negative implications, companies and other key stakeholders can identify various initiatives that will minimize every anticipated negative impact. Motoori, McLellan, and Tezuka (2018) encourage mining corporations to diversify their models and consider the importance of recycling existing materials or metals. This approach is sustainable and capable of reducing the dangers of mining. Governments can also formulate and implement powerful policies that compel different companies to engage in desirable practices, minimize pollution, and reduce noise pollution. Such guidelines will make sure that every company remains responsible for remediating their sites. Mensah et al. 2015) also support the introduction of laws that compel organizations to conduct environmental impact assessment analyses before starting their activities. This model will encourage them to identify regions or sites that will have minimal effects on the surrounding population or aquatic life. The concept of green mining has emerged as a powerful technology that is capable of lessening the negative implications of mining. This means that all activities will be sustainable and eventually meet the diverse needs of all stakeholders, including community members. Finally, new laws are essential to compelling companies to shut down and reclaim sites that are no longer in use.
Extraction from the Ore Body
Copper mining is a complex process since it is found in more stable forms, such as oxide and sulfide ores. These elements are obtained after the overburden has been removed. Corporations complete a 3-step process or procedure before obtaining pure copper. This is usually called ore concentration, and it follows these stages: froth flotation, roasting, and leaching (Sikamo, Mwanza & Mweemba 201). During froth flotation, sulfide ores are crushed to form small particles and then mixed with large quantities of water. Ionic collectors are introduced to ensure that CuS becomes hydrophobic in nature. The introduction of frothing agent results in the agitation and aeration of the slurry (Sikamo, Mwanza & Mweemba 2016). This means that the ore containing copper will float to the surface. All tailings will sink to the bottom of the solution. The refined material can then be skimmed and removed.
The next stage is that of roasting, whereby the collected copper is baked. The purpose of this activity is to minimize the quantities of sulfur. Such a procedure results in sulfur dioxide, As, and Sb (Yaras & Arslanoglu 2017). This leaves a fine mixture of copper and other impurities. The next phase of the ore concentration method is that of leaching. Different Compounds are used to solubilize the compound, such as H2SO4 and HCI. The leachate will then be deposited at the bottom and purified.
Smelting is the second stage that experts use to remove copper from its original ore. This approach produces iron and copper sulfides. Exothermic processes are completed to remove SiO2 and FeSiO3 slag (Yaras & Arslanoglu 2017). According to this equation, oxygen is introduced to produce pure copper and sulfur dioxide:
CuO + CuS = Cu(s) + SO2
The final phase is called refinement. The collected Cu is used as anodes and cathodes, whereby they are immersed in H2SO4 and CuSO4. During this process, copper will be deposited on the cathode while the anode will dissolve in the compound. All impurities will settle at the bottom (Sikamo, Mwanza & Mweemba 2016). From this analysis, it is notable that a simple process is considered to collect pure copper from its ore body.
How Copper Mining Impacts the Environment
Copper mining is a complex procedure that requires the completion of several steps if a pure metallic compound is to be obtained. This means that it is capable of presenting complicated impacts on the natural environment. Copper mining can take different forms depending on the location of the identified ores and the policies put in place in the selected country (Yaras & Arslanoglu 2017). Nonetheless, the entire process will have detrimental effects on the surrounding environment. Due to the intensity of operations and involvement of heavy machinery, this process results in land degradation. The affected regions will have huge mine sites that disorient the original integrity of the environment.
Since copper is one of the most valuable metals in the world today due to its key uses, many companies continue to mine it in different countries. This practice has triggered the predicament of deforestation (Sikamo, Mwanza & Mweemba 2016). Additionally, rainwater collects in abandoned mine sites or existing ones, thereby leaking into nearby rivers, boreholes, or aquifers. This means that more people are at risk of being poisoned by this compound.
Air pollution is another common problem that individuals living near copper mines report frequently. This challenge is attributable to the use of heavy blasting materials and machinery. The dust usually contains hazardous chemicals that have negative health impacts on communities and animals. Some of the common ailments observed in most of the affected regions include asthma, silicosis, and tuberculosis (Mensah et al., 2015). This challenge arises from the toxic nature of high levels of copper. These problems explain why companies and stakeholders in the mining industry should implement superior appropriate measures and strategies to overcome them. Such a practice will ensure that they meet the needs of the affected individuals and make it easier for them to pursue their aims.
Copper processing can have significant negative implications on the integrity of the environment. For instance, the procedure is capable of producing tailings and overburden that have the potential to contaminate different surroundings. According to Mensah et al. (2015), some residual copper is left in the environment since around 85 percent of the compound is obtained through the refining process. This means that it will pose health problems to people and aquatic life. Other metals are present in the produced tailings, such as iron and molybdenum. During the separation process, hazardous chemicals and gases will be released, such as sulfur dioxide. This is a hazardous compound that is capable of resulting in acidic rain, thereby increasing the chances of environmental degradation.
There are several examples that explain why copper is capable of causing negative impacts on the natural environment. For example, Queenstown in Tasmania has been recording large volumes of acidic rain (Mensah et al., 2015). This is also the same case for El Teniente Mine in Chile. Recycling and reusing copper can be an evidence-based approach for minimizing these consequences and maintaining the integrity of the environment.
Farmlands that are polluted with this metal compound will have far-reaching impacts on both animals and human beings. This is the case since the absorption of copper in the body can have detrimental health outcomes. This form of poisoning can disorient the normal functions of body organs and put the individual at risk of various conditions. People living in areas that are known to produce copper continue to face these negative impacts (Yaras & Arslanoglu 2017). Such challenges explain why a superior model is needed to overcome this problem and ensure that more people lead high-quality lives and eventually achieve their potential.
The above discussion has identified mining as a major economic activity that supports the performance and integrity of many factories, countries, and companies. However, this practice continues to affect the natural environment and making it incapable of supporting future populations. Mining activities result in deforestation, land obliteration, air pollution, acidic rain, and health hazards. The separation of copper from its parent ore is a procedure that has been observed to result in numerous negative impacts on the environment and human beings. These insights should, therefore, become powerful ideas for encouraging governments and policymakers to implement superior guidelines that will ensure that miners minimize these negativities by remediating sites.
Mensah, AK, Mahiri, IO, Owusu, O, Mireku, OD, Wireko, I & Kissi, EA 2015, ‘Environmental impacts of mining: a study of mining communities in Ghana’, Applied Ecology and Environmental Sciences, vol. 3, no. 3, pp. 81-94.
Minerals Council of Australia 2019, Australian minerals , Web.
Motoori, R, McLellan, BC & Tezuka, T 2018, ‘Environmental implications of resource security strategies for critical minerals: a case study of copper in Japan’, Minerals, vol. 8, no. 12, pp. 558-586.
Sikamo, J, Mwanza, A & Mweemba, C 2016, ‘Copper mining in Zambia – history and future’, The Journal of the South African Institute of Mining and Metallurgy, vol. 116, no. 1, pp. 491-496.
Yaras, A & Arslanoglu, H 2017, ‘Leaching behaviour of low-grade copper ore in the presence of organic acid’, Canadian Metallurgical Quarterly, vol. 57, no. 3, pp. 319-327.
- Chicago (A-D)
- Chicago (N-B)
IvyPanda. (2024, February 22). Mining and Its Impact on the Environment. https://ivypanda.com/essays/mining-and-its-impact-on-the-environment/
"Mining and Its Impact on the Environment." IvyPanda , 22 Feb. 2024, ivypanda.com/essays/mining-and-its-impact-on-the-environment/.
IvyPanda . (2024) 'Mining and Its Impact on the Environment'. 22 February.
IvyPanda . 2024. "Mining and Its Impact on the Environment." February 22, 2024. https://ivypanda.com/essays/mining-and-its-impact-on-the-environment/.
1. IvyPanda . "Mining and Its Impact on the Environment." February 22, 2024. https://ivypanda.com/essays/mining-and-its-impact-on-the-environment/.
Bibliography
IvyPanda . "Mining and Its Impact on the Environment." February 22, 2024. https://ivypanda.com/essays/mining-and-its-impact-on-the-environment/.
- The Importance of Copper in the 21st Century
- How is Aluminium Ore Converted to Aluminium Metal?
- Copper Mining in Economy, Environment, Society
- Physical and Chemical Properties of Copper
- Water and Energy Problems in Mining Industry
- Gold and Copper Market and Industry Overview
- Iron: Properties, Occurrence, and Uses
- Fortescue Metals Group (FMG) and the Yindjibarndi Aboriginal Land
- Hydrated Copper (II) Sulphate Experiment
- The Ballpoint Pen: Materials, Process and Issues
- Wind Power in West Texas and Its Effects
- National Park Utah: Situation at Arches
- Green Revolution in the Modern World
- Project Oxygen: Making Management Matter
- The Ongoing Problem of Lead in Drinking Water in Newark, New Jersey
Thank you for visiting nature.com. You are using a browser version with limited support for CSS. To obtain the best experience, we recommend you use a more up to date browser (or turn off compatibility mode in Internet Explorer). In the meantime, to ensure continued support, we are displaying the site without styles and JavaScript.
- View all journals
- My Account Login
- Explore content
- About the journal
- Publish with us
- Sign up for alerts
- Open access
- Published: 08 March 2023
Reshoring silicon photovoltaics manufacturing contributes to decarbonization and climate change mitigation
- Haoyue Liang 1 &
- Fengqi You ORCID: orcid.org/0000-0001-9609-4299 1 , 2 , 3
Nature Communications volume 14 , Article number: 1274 ( 2023 ) Cite this article
12k Accesses
12 Citations
184 Altmetric
Metrics details
- Climate-change impacts
- Solar cells
The globalized supply chain for crystalline silicon (c-Si) photovoltaic (PV) panels is increasingly fragile, as the now-mundane freight crisis and other geopolitical risks threaten to postpone major PV projects. Here, we study and report the results of climate change implications of reshoring solar panel manufacturing as a robust and resilient strategy to reduce reliance on foreign PV panel supplies. We project that if the U.S. could fully bring c-Si PV panel manufacturing back home by 2035, the estimated greenhouse gas emissions and energy consumption would be 30% and 13% lower, respectively, than having relied on global imports in 2020, as solar power emerges as a major renewable energy source. If the reshored manufacturing target is achieved by 2050, the climate change and energy impacts would be further reduced by 33% and 17%, compared to the 2020 level. The reshored manufacturing demonstrates significant progress in domestic competitiveness and toward decarbonization goals, and the positive reductions in climate change impacts align with the climate target.
Similar content being viewed by others
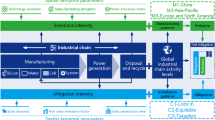
Deploying solar photovoltaic energy first in carbon-intensive regions brings gigatons more carbon mitigations to 2060
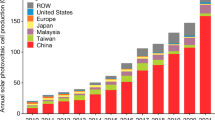
Quantifying the cost savings of global solar photovoltaic supply chains
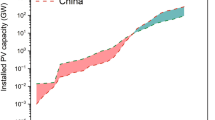
Analogical environmental cost assessment of silicon flows used in solar panels by the US and China
Introduction.
At the 2021 United Nations Climate Change Conference (COP 26) held in the U.K., attending countries agreed to sustain the goal of limiting global temperature rises to 1.5 degrees and to finalize the unfinished elements of the Paris Agreement 1 , 2 , 3 , 4 . The U.S. is ramping up its actions to create a carbon-free power sector by 2035 and to achieve “net-zero” greenhouse gas (GHG) emissions by 2050 5 . Facilitating this degree of climate change mitigation will require the accelerated deployment of fundamentally clean technologies, including sustainable solar photovoltaic (PV) technology, which marks the cleanest and cheapest form of electricity 6 . The growth of solar power has increased exponentially from small-scale applications to one of the mainstream sources of electricity, growing by more than 20% per year over the past six years 7 , 8 . The U.S. installed 14.9 GW AC (19.2 GW DC ) of PV in 2020, with PV accounting for approximately 40% of new electricity generation, up from just 4% in 2010 9 , 10 . By 2035, solar power is projected to support 40% of U.S. electricity demand, a tenfold increase over the solar output in 2021 11 . By 2050, solar could be nearly half of the electricity supply 11 . Solar power usage in the U.S. is also expected to reach 1067 TWh, 720% above the 130 TWh usage in 2020 8 . Following these projections, the market supply of PV technologies will be driven by energy policy goals and the aggressive pace of PV market demand.
The energy policy goals and the soaring PV panel demand impose a great supply challenge for the PV industry to catch up with the growing needs in the coming decades. Most U.S. PV installations relied on the use of imported panels, mainly coming from Asia 12 . Safeguard tariffs were placed onto imported silicon solar modules and applied for four years starting from 2018, with the duties declining 5% each subsequent year to arrive at 15% in the fourth year 6 , as part of a trend of trade protectionism regarding PV manufacturing. Around 96% of the world’s PV production comes from crystalline silicon (c-Si), which includes single-crystalline silicon (sc-Si), ribbon silicon (r-Si), and multi-crystalline silicon (mc-Si), representing the most widely used semiconducting materials 11 , 13 , 14 . While U.S. dependence on imported c-Si PV modules is non-negligible, the market favorability for PV imports is declining, as revealed by a decreasing fraction of imported PV panels despite a decrease in the tariff on imported silicon solar cells in recent years 11 , 12 .
Both supply instability and temporary trade restrictions due to geopolitical issues are the reasons behind the shift away of manufacturing from foreign supplies 15 . The effects of supply chain restructuring continue to propagate throughout the PV industry, as the overreliance on global trade structure emphasizes disruptions that jeopardize all countries involved 11 , 16 . While various countries locked their borders and international freighting took a nosedive in recent years due to the pandemic 17 , the demand for solar panels remains high due to elevated renewable electricity demand. Significant challenges have emerged in solar panel supply chains that face increasing risk from relying on external imports, which respond sensitively to any freight crisis or other potential disruptions 16 . Manufacturers want to move away from offshoring due to supply chain woes, geopolitical instability, freight cost, and many other considerations 18 .
The production of solar panels is expected to defer to more domestic sourcing, emphasizing self-sufficiency 11 , driven by growing geopolitical concerns and rapidly evolving trade relationships. Furthermore, reshored manufacturing facilitates close proximity to customers and markets, provides positive impacts on the domestic economy, and is also encouraged by policies and incentives 18 . The Inflation Reduction Act provides the necessary impetus for the domestic solar product manufacturing industry to catch up with countries that have outpaced the U.S. in clean energy technological development, innovation, and production 19 . The manufacturing tax incentives and investments, coupled with domestic content credits and the robust “Made in America” agenda, will provide the U.S. with the tools necessary to integrate climate and economic goals into multilateral trade discussions 20 . Past studies have focused on how reshored manufacturing could enhance solar PV product development, delivery performance, and cost leadership 21 , and the importance of the operation facilities in the PV panel reshoring decisions 22 . Despite the many existing studies related to reshored PV panel manufacturing decisions, the climate implications arising from the ongoing and upcoming solar panel value chain restructuring have not been systematically addressed with first-order analyses or otherwise been understood comprehensively. Whether reshored c-Si PV panel manufacturing, foremost a strategy to withstand supply disruptions, truly aligns with the ongoing climate target and energy policy to additionally accomplish climate change mitigation and energy consumption reduction remains a knowledge gap.
In this study, we perform the analysis to clarify the energy and environmental impacts of bringing c-Si PV production back to the U.S. by comparing the offshore (outsourced) manufacturing cases from 2010 to 2020 and the reshored (domestic) manufacturing scenarios from 2020 to 2050. To unilaterally quantify the impact of reshoring, we study the climate change impact of the hypothetical reshored scenario in 2020 and compare it to the offshore case in the same year. We also interpret the climate implications of a delayed reshoring schedule. To understand how the expansion of the solar panel industry that already greens the power grid could further promote climate change mitigation during its manufacturing stages together with the power of reshoring, we forecast reshored PV panel manufacturing scenarios with renewable penetration to the energy supply. The renewable penetration is also only made possible with the growing supply and demand of solar PV panels, as the ambitious outline would see solar electricity supply rising to 40% by 2035 before ultimately hitting 45% by 2050 11 . The prospective assessment of the decarbonization and climate change implications of reshored c-Si PV panel manufacturing in the U.S. to alleviate the supply chain woes emerging from unpredictable disruptions and geopolitical concerns is the major focus of this work. A comprehensive quantitative analysis of the detailed contribution of reshored manufacturing, renewable penetration as a result of solar PV industry growth, as well as other technological advancements in climate change mitigation and energy consumption reduction is provided. This work sheds light on some major policy implications. The implication of a positive reduction in climate impacts provides policymakers with a big picture conclusion that reshored manufacturing of PV panels aligns with the energy policy goals and contributes to the climate targets.Domestic c-Si PV panel manufacturing leads to a 23% reduction in climate change impact and a 4% decline in energy use from panel production compared to outsourced manufacturing because of reshoring. Bringing manufacturing back home helps the PV panel industry realize the decarbonization goal. If reshored PV manufacturing is achieved by 2035, the estimated GHG emissions and energy consumption from panel production would be 30% and 13% lower, respectively, than having relied on trading partners as in 2020. If the reshored manufacturing target is met by 2050, the climate impacts and energy use would then be reduced by 33% and 17%, as solar PV emerges as a major power source that characterizes the energy market for the remainder of the 21 st century. Domestic sourcing at a later date also aligns with the climate target and energy policy goals.
Results and discussion
Reshored c-Si PV manufacturing tackles logistic challenges, but whether it directly reduces GHG emissions and energy use has not yet been discovered based on quantitative analysis. Exploring the climate change and energy impacts help us understand if reshored manufacturing aligns with the climate target. We perform a comparative and prospective life cycle assessment (LCA) study of several reshored manufacturing scenarios and outsourced manufacturing cases to examine the energy and climate impacts of fully eliminating dependence on foreign PV supplies. We define three cases (2010, 2015, 2020 offshore manufacturing) and seven scenarios (2020, 2025, 2030, 2035, 2040, 2045, 2050 reshored manufacturing). The reshored scenario in 2020 is studied to examine the climate impacts of solely bringing manufacturing back to the U.S. by comparing it with the outsourced manufacturing case in 2020. Moreover, reshored scenarios from 2025 to 2050 in 5-year increments are forecasted with cleaner power compositions such as wind, solar, geothermal, etc., building up from 21% renewable power contribution in 2020 to 42% in 2050 8 . We project and examine future scenarios spanning a wide range of time points from the near term to mid-century because of the potential uncertainties regarding the speed of domestic PV production scaling and the rate of equipment and workforce training expansion. Past studies expressed concern that trade restrictions and emphases on reshoring might slow the adoption of sustainable energy technologies, and the U.S. might not be fully equipped for rapidly upscaling domestic solar panel production 23 , 24 . Trade wars may also affect the environment by altering the global supply and consumption systems 25 , 26 , which become less conducive for less-developed regions to transition to clean energy 27 . Manufacturing efforts face an unpredictable future, and uncertainties remain regarding exactly when the reshoring of PV panels can be accomplished due to trade barriers, financing problems, workforce limitations, and so on. That is why multiple future reshored manufacturing scenarios at different time points, ranging from 2025 to 2050, are included in this study. These projections of different reshoring levels at various time points can be regarded as a sensitivity analysis to incorporate the temporal variations for when reshoring can be achieved. We study reshored manufacturing scenarios because legislations not only include targeted tax incentives aimed at manufacturing U.S.-sourced solar materials but also include key requirements around domestic sourcing. The Inflation Reduction Act opens up an opportunity for spurring U.S. solar technology supply chain as countries around the world race to lead the clean energy economy 28 , 29 . Reasonable predictions for these scenarios are made regarding the U.S.-centered domestic supplies as we foresee opportunities to grow a competitive supply chain of module components in regions like Alabama, Florida, Georgia, and so on 30 . We also study outsourced manufacturing cases in 2010 and 2015 to understand the impacts of the ever-changing global PV module supply chain structure on decarbonization.
For PV power plants, the majority of GHGs are emitted upstream of module manufacturing 31 . Solar panels do not produce emissions while generating electricity, but the operations and maintenance life cycle stage and the end-of-life treatment stage are included in this study to emphasize the relative emission reductions from panel manufacturing reshoring in the context of PV panel lifetime emissions. The operation and maintenance life cycle phase involves tasks like module cleaning, preventive maintenance (such as replacing inverters), as well as the repair of broken components, and the end-of-life treatment stage involves dismantling and shredding solar panels 32 . Based on the best available data 33 , 34 , a 1 m 2 PV panel emits 0.27 kg CO2 eq GHG and demands 48 MJ of energy during its use stage, and emits 0.57 kg CO 2 eq GHG and demands 74 MJ of energy at its end-of-life treatment stage. The energy and environmental impacts of the operations and maintenance life cycle stage and the end-of-life treatment stage can be useful to understand the relative emission reductions from PV panel manufacturing in the overall context of PV panel life cycle.
Reshoring as a decarbonization strategy
In this study, climate change mitigation potential and energy performance of PV panel manufacturing are presented to study the energy and decarbonization impacts of reshoring on solar panel production. The quantitative analysis is conducted based on two important climate-related metrics, global warming potential (GWP) and cumulative energy demand (CED) 35 . To investigate the impact of switching from offshore manufacturing to domestic production on the c-Si PV panels, we compare the reshored scenario (Fig. 1b , d , f ) in 2020 with the outsourced case (Fig. 1a , c , e ) in the same year. Figure 1a , c , and e present the GHG emissions for production across the entire supply chain for each trading partner (China, Malaysia, Japan, etc.) from silica sand production to panel manufacturing between 2010 and 2020 for three types of c-Si materials, and emissions from shipping of these panels to the U.S. are also included. Figure 1b , d , and f showcase the emissions from U.S. domestic production of PV panels from 2020 to 2050 in five-year increments. Compared with relying on global supplies (offshore case) in 2020, domestic manufacturing of c-Si PV modules in the U.S. reduces GHG emissions by 23% and energy use by 4%. The offshore case in 2020 mainly relied on supplies from Malaysia (38%), Vietnam (21%), Thailand (17%), South Korea (9%), China (6%), and Singapore (3%) 36 , 37 . Manufacturing PV panels in Malaysia under the 2020 offshore case generates 42% more GHG emissions than manufacturing in the U.S., mainly due to the high emissions (26%-29% of all emissions) from solar grade silicon manufacturing stage for all three types of c-Si technologies (Fig. 1a , c , e ), and the sc-Si crystal production stage that generates 26% to 28% of all emissions for sc-Si technology (Fig. 1a ).

Results are presented for ( a ) single-crystalline silicon (sc-Si) PV in offshore cases, ( b ) sc-Si PV in reshored scenarios, ( c ) ribbon silicon (r-Si) in offshore cases, ( d ) r-Si in reshored scenarios, ( e ) multi-crystalline silicon (mc-Si) in offshore cases, as well as ( f ) mc-Si in reshored scenarios. Reshored manufacturing scenarios in ( b , d , and f ) illustrate the downward climate change impact trend over time, whereas offshore manufacturing cases in ( a , c , and e ) do not guarantee climate change mitigation over time, as illustrated by the higher emissions in 2015 than in 2010 in some regions. To study the impact of reshoring in 2020, we compare the 2020 case and the 2020 scenario. The sources of PV supplies in the 2020 case include China, South Korea, Malaysia, Singapore, Thailand, and Vietnam, as shown in ( a , c , and e ). The PV panels in the 2020 scenario are only manufactured in the U.S., as shown in ( b , d , and f ). We see a reduction of 23% global warming potential from PV panel manufacturing on average as a result of reshoring.
Although the Malaysian government launched its Green Technology Policy in July 2009 to encourage and promote the use of renewable energy for Malaysia’s sustainable development 38 , almost half of its power generation still relied on coal (46%) a decade later after the policy was launched 39 . On the other hand, the U.S. relied heavily on natural gas (39%), which contributes almost twice as much to total electricity generation as coal source (20%) 39 . Besides the quartz mining stage, all stages require the use of electricity, as shown in Fig. 2 . Among them, more high-voltage electricity power is needed in solar grade silicon manufacturing stage, which is on average six to ten times the amounts needed for electronics grade silicon and metallurgical grade silicon production. The power sector is one of the major sources of GHG emissions. The differences in power mixes between countries lead to discrepancies in climate change impacts of silicon manufacturing, which directly results in the gap in GHG emissions between the outsourced case and domestic scenario in 2020.
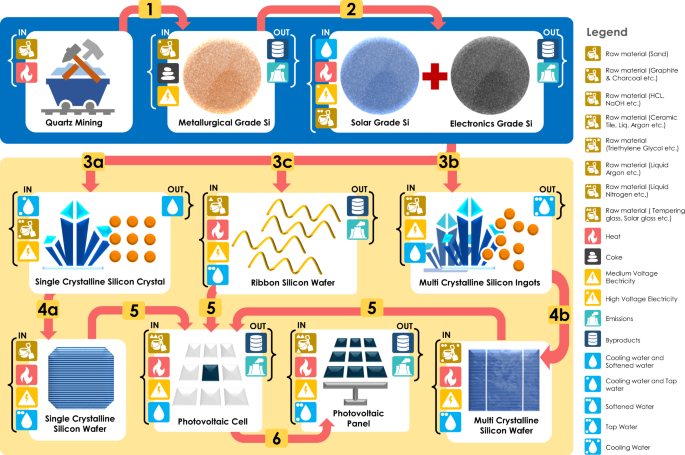
The output from quartz mining stage is the silica sand, which is the input for the metallurgical grade silicon production (step 1). Metallurgical grade silicon is the input of both solar grade silicon production and electronics grade silicon production (step 2). Three types of crystalline silicon materials go through different paths for wafer production (step 3a & 4a for single-crystalline silicon, step 3b & 4b for multi-crystalline silicon, and step 3c for ribbon silicon). Photovoltaic cells are made from wafers (step 5), and photovoltaic panels are made from cells (step 6).
Similarly, the energy performance of offshore cases (Fig. 3a , c , e ) and reshored scenarios (Fig. 3b , d , f ) are presented. Figure 3a , c , and e , similar with Fig. 1a , c , and e , exhibit the sources of PV supplies in cases from 2010 to 2020 across the entire supply chain for each trading partner (China, Malaysia, Japan, etc.), while Fig. 3b , d , and f showcase the scenarios in which production occurs in the U.S. itself. We see a 4% reduction in energy consumption when switching from offshore to reshored manufacturing in the same year, despite the decline in energy use being less significant than that of climate change mitigation. As opposed to the climate change impacts of reshored manufacturing in the U.S., which always results in lower GHG emissions than all offshore suppliers (30% lower than China, 17% lower than South Korea, 3% lower than Singapore, 18% lower than Thailand, as shown in Fig. 1 ), the energy consumption in the U.S. in the reshored scenario is not always lower than all suppliers in the offshore case in 2020, as shown in Fig. 3 . Specifically, manufacturing c-Si PV in the U.S. requires more energy use than some of the suppliers in the outsourced case, such as Singapore (2% lower than the U.S.), Thailand (1% lower than the U.S.), and Vietnam (5% lower than the U.S.) (Fig. 3 ).
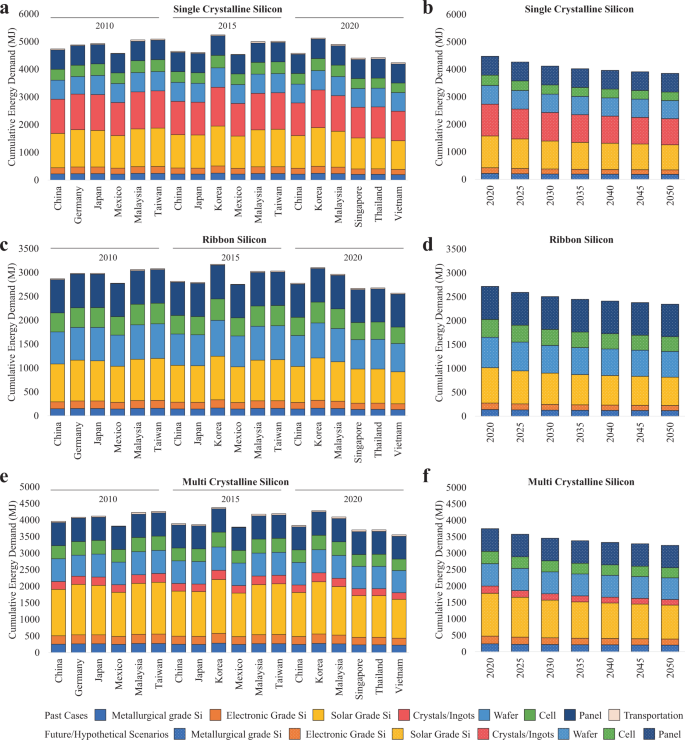
Results are presented for ( a ) single-crystalline silicon (sc-Si) PV in offshore cases, ( b ) sc-Si PV in reshored scenarios, ( c ) ribbon silicon (r-Si) in offshore cases, ( d ) r-Si in reshored scenarios, ( e ) multi-crystalline silicon (mc-Si) in offshore cases, as well as ( f ) mc-Si in reshored scenarios. Reshored manufacturing scenarios in ( b , d , and f ) illustrate the downward energy consumption trend over time, whereas offshore manufacturing cases in ( a , c , and e ) show that energy consumption can be higher when supplies change, as illustrated by the higher energy use in 2015 of some suppliers than in 2010. To study the impact of reshoring in 2020, we compare the 2020 case and the 2020 scenario. The sources of PV supplies in the 2020 case include China, South Korea, Malaysia, Singapore, Thailand, and Vietnam, as shown in ( a , c , and e ). The PV panels in the 2020 scenario are only manufactured in the U.S., as shown in ( b , d , and f ). We see a reduction of 4% energy use from PV panel manufacturing on average as a result of reshoring.
We explore the reasons behind the lower energy footprint of manufacturing PV panels in offshore cases. Vietnam relied heavily on hydropower (28%), which is a much more efficient way to generate electricity than coal and natural gas. Modern hydro turbines can convert as much as 90% of energy into electricity, whereas the best fossil fuel plants are only 50% efficient 40 . Thus, less energy is required to manufacture PV panels in Vietnam due to more efficient energy usage. Meanwhile, transportation accounts for just 1% of CED, a minimal amount compared to the manufacturing stages. After oceanic shipping (1% of CED) is included for supplies from Vietnam, the total energy usage is still 5% lower than reshored manufacturing in the U.S. Despite the similar energy performance of production in Thailand and Singapore which also relied heavily on natural gas, as well as the lower energy use of c-Si PV production in Vietnam, the offshore manufacturing case still results in 4% higher CED compared with the reshored scenario in the same year. As mentioned earlier, the U.S. only attributed 41% of its PV supplies from these three countries in 2020 (21% from Vietnam, 17% from Thailand, and 3% from Singapore); 36 the weighted average energy use when the U.S. relied on foreign suppliers is still higher than domestic production. However, reshored manufacturing does not guarantee an absolute energy performance advantage compared to offshore manufacturing due to the proximity (4% variation) of energy consumptions under the reshored scenario and the weighted average outsourced case.
Future reshored manufacturing scenarios with renewable penetration to the power grid
Meeting the increasing demand for green power worldwide, growing shares of renewable energy sources over time as well as switching from global sourcing to reshored manufacturing would lead to greater climate change mitigation from c-Si PV module production in the future. The growing shares of renewable energy sources will not be possible without the increasing demand and supply of c-Si PV panels. Renewable penetration and expansion of c-Si PV panel manufacturing facilitate each other to achieve climate benefits. The amount of GHG emissions generated from reshored c-Si PV module production in the U.S. in 2050 is anticipated to reduce by 33% compared to relying on foreign supply in 2020 and 30% lower in 2035 than in 2020 (Fig. 1 ). The forecasted significant climate change mitigation is fulfilled by both reshoring manufacturing back to the U.S. and the large renewable penetration to the power grid, which is anticipated to happen in the U.S. in the next few decades 41 . The usage of renewable energy, including wind, solar, geothermal, etc., contributes to a 470% to 520% greater fraction of energy in 2050 than in 2010, exemplifying the far-reaching impacts of penetration of renewables into the power mix on CED impact analysis 8 . Compared to 2020, the coal-sourced share of electricity generation in the U.S. is projected to decrease by 18% in 2030, 33% in 2040, and 43% in 2050, while the nuclear source share would decrease by 27% in 2030, 37% in 2040, and 44% in 2050 8 , as shown in Supplementary Methods 2 : Electricity mix. As the U.S. transitions to greener sources of electricity, it is projected to rely on wind nearly twice as much starting merely from 2024, compared to the 2020 level. Among the renewable fuels, solar power is anticipated to increase by eightfold from 132 billion kWh in 2020 to 1071 billion kWh forecasted in 2050 8 (Supplementary Fig. 11 ). GHG emissions decrease appreciably over time as a result of both reshoring and the progression to more renewable power generation sources as a result of reshoring.
Despite the climate change mitigation, our results also shed light on the significant energy performance improvements. Compared with relying on global supplies in 2020, we project that domestic manufacturing of c-Si PV modules in the U.S. in 2035 and 2050 requires 13% and 17% less CED (Fig. 3 and Fig. 4 ), including 32% less non-renewable fossil energy (Fig. 4 ), indicating a significant energy reduction trend resulting from supply transition. Based on the projections on the energy decarbonization transition that happens alongside reshoring, we see not only larger shares of renewables accounting for primary energy consumption but, resultantly, overall lower primary energy consumptions over the years for all c-Si technology, as shown in Fig. 4 .
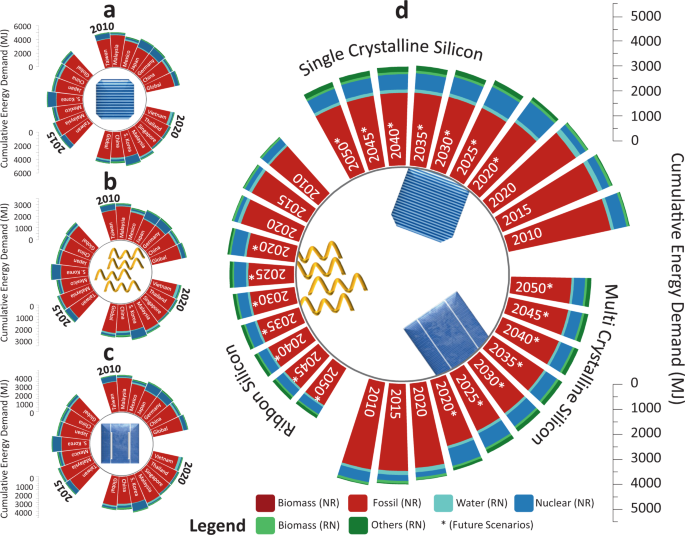
Three cases in 2010, 2015, and 2020 are presented based on three types of crystalline silicon photovoltaic technologies: ( a ) single-crystalline silicon, ( b ) ribbon silicon, ( c ) multi-crystalline silicon. a – c represent the energy use for production across the entire supply chain for each trading partner. Seven scenarios in 2020, 2025, 2030, 2035, 2040, 2045, and 2050 are presented altogether in ( d ), with * indicating future scenarios.
Past offshore manufacturing cases
The trade structure significantly changed over the past few years, leading to an increased GHG emission from 2010 to 2015 (Fig. 1 ). The U.S. mainly relied on PV supplies from Taiwan (41%), Malaysia (29%), China mainland (14%), Germany (6%), Japan (6%), and Mexico (2%) in 2010, and Malaysia (32%), China mainland (31%), Taiwan (7%), Japan (6%), and Mexico (5%) in 2015, as shown in Fig. 5 . The supply share of the PV system in Taiwan drastically reduced from 41% to 7%, and that of China mainland went up from 14% to 31% from 2010 to 2015, while other regions’ shares changed over time but not to any extent that would drive significant impacts (Fig. 5 ).
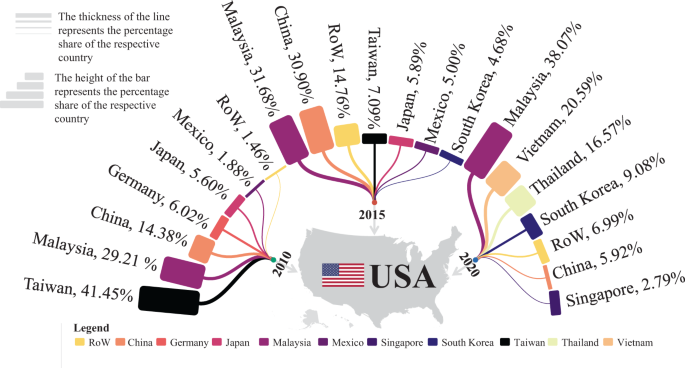
The U.S. mainly imported solar photovoltaic products from Asian trading partners. The market shares of module supplies changed dramatically over the years due to geopolitical tensions, safeguard tariffs, policies, etc. The rest of the world (RoW) includes all other suppliers other than the top six suppliers.
The GHG emissions from panel production of the 2015 outsourced case are estimated to be even higher than the 2010 outsourced case. Although the life cycle carbon footprint of PV systems in China decreases by 5% (20 kg CO 2 equivalent per 1 m 2 of PV module manufactured) in 2015 compared to 2010, the U.S. imported more panels from mainland China in 2015 (31%) than in 2010 (14%). The GHG emissions from PV panel production in mainland China in 2015 are lower than in mainland China in 2010, but are still higher than the other suppliers (Germany, Japan, Mexico, Malaysia, Taiwan) in 2010, as shown in Fig. 1a , c , e , leading to a higher weighted sum of emissions from trading partners in 2015 than in 2010. Therefore, offshore manufacturing does not guarantee decreasing GHG emissions over time. The fluctuating GWP over the years is highly relevant to the displacements in the importing supply share of c-Si PVs. A supply chain crisis can occur at anytime that threatens the growth of the solar energy and the PV industry or even leads to increased GHG emissions in c-Si PV manufacturing. To stabilize the supply and to attain consistent emission reduction, reshoring is an option to consider, and growing efforts of reshoring manufacturing have been demonstrated 5 .
Similarly, the energy consumption of manufacturing PV panels in 2015 in some countries is higher than in the 2010 outsourced case. For instance, South Korea was not a major source of PV supplies to the U.S. in 2010, but it became one of the top six suppliers in 2015. Manufacturing PV panels in South Korea in 2015 requires 5% more energy than the average 2010 case, while Malaysia and Vietnam in 2015 requires 2% to 10% more energy than most suppliers (China, Germany, Japan, Mexico) in 2010. This indicates that when manufacturing locations shift from China, Germany, Japan, and Mexico in 2010 to South Korea, Malaysia, and Vietnam in 2015, the energy usage increases, as shown in Fig. 3 . Clearly, with the ever-evolving pace of imported freight, the future of worldwide module production and the PV supply chain is uncertain, just as it was so easily disrupted by the supply crisis due to the COVID-19 pandemic 42 . Since the U.S. economy has faced many supply bottlenecks that contribute to high inflation, a strategy that ensures domestic manufacturing in the U.S. is encouraged 43 . As the U.S. PV demand growth continues, there might be an opportunity for further domestic manufacturing expansion, particularly given the potential supply chain disruption 11 .
Contributors to the climate of reshoring PV manufacturing
Together with the impacts from reshoring and renewable penetration, we incorporate other factors with temporal or geographical variations, such as module efficiency, performance ratio, solar irradiation, and grid efficiency, in our analysis to study the energy and environmental impacts of these factors considered. Based on the Parameters under “ Methods ”, we estimate the carbon emission factor and the energy payback time (EPBT) of outsourced cases and domestic scenarios. We see that while other factors are taken into consideration, as illustrated in Fig. 6 , the estimations of these two metrics also differ drastically between cases that rely on foreign supply and scenarios that depend on domestic production. As it stands, reshoring PV panel manufacturing sees a drastic reduction of carbon emission factor of 31% in 2035 and 33% in 2050 and EPBT decline of 14% in 2035 and 17% in 2050, compared with the 2020 offshore case (Fig. 6 ). The reductions can chiefly be accredited to the switch to reshored manufacturing and the changing breakdown of energy sources in the U.S. If reshored manufacturing can be achieved in 2035, among 31% of the reduction in carbon emission factor, reshoring leads to 23% of emission factor decrease while renewable penetration to the power grid contributes to 8%. On the other hand, when it comes to reductions in EPBT, 4% of EPBT decline is attributed to reshoring, while the remaining 10% are the credits of renewable penetration. We see that the act of reshoring has a greater impact on carbon emission reduction. The renewable penetration anticipated in the U.S. has a more significant influence on EPBT, but the projections on renewable penetration, including the soaring solar energy, can only be implemented if the PV panel supply surge in the next few years. Therefore, renewable penetration and reshored manufacturing for increasing PV demand are not mutually exclusive but rather in the same boat. They work together to drop carbon emission factors and lower EPBT of manufacturing c-Si PV panels.
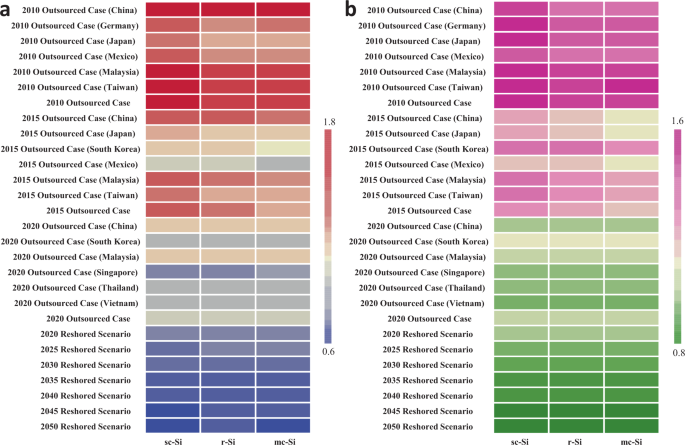
The switch to reshored manufacturing and increasing renewable penetration to the power grid work together to drop ( a ) carbon emission factor and lower ( b ) energy payback time (EPBT) of manufacturing c-Si PV panels. The presented results are normalized and vary based on the colors shown on the color bar. In particular, the red and pink colors represent high carbon emission factors and high EPBT, suggesting more energy and environmental burdens, while the blue and green colors represent low carbon emission factors and low EPBT, indicating lower burdens. The 2020 outsourced case is defined as the reference for normalization.
Sensitivity analysis
Besides our main analysis, we also study how other factors can have an impact on the energy and climate change profiles. We perform sensitivity analysis of various parameters on CED, GWP, carbon emission factor, EPBT, and energy return on energy invested (EROI) estimations. We first explore whether choosing the top six suppliers to represent the trading partners would or would not lead to significant bias in the energy and climate change results. As an additional litmus test for the number of global PV manufacturing locations to represent offshore locations, to ensure that the six suppliers chosen to represent the global supply sourcing market are appropriate, we conducted a sensitivity analysis on the number of suppliers designated in the market share calculations. We perform a sensitivity analysis specifically for the 2015 offshore case since the global supply of c-Si PV to the U.S. is less distinctly dominated by the top six suppliers (85% of supplies come from the top six) in 2015, whereas it was well-dominated by the top six in 2010 (99% from top six) and 2020 (93% from top six), as shown in Fig. 5 . To perform this sensitivity analysis that includes more spatial variation, we study and compare the energy and environmental impacts of the top nine suppliers. Alongside the top six locations, we include Germany (4.07%), Singapore (3.16%), and Vietnam (2.90%) as the top suppliers. The calculated energy profile represented by CED decreases by less than 1% (0.97% for sc-Si, 0.89% for r-Si, and 0.94% for mc-Si), while environmental impact represented by GWP decreases by less than 2.5% (2.44% for sc-Si, 2.32% for r-Si, and 2.31% for mc-Si), as shown in Fig. 7a . The results thereby affirm that designating just the top six exporters as the major market share components is a succinct yet sufficiently representative group by which to assess the c-Si PV supply chain and trade structure before 2020.
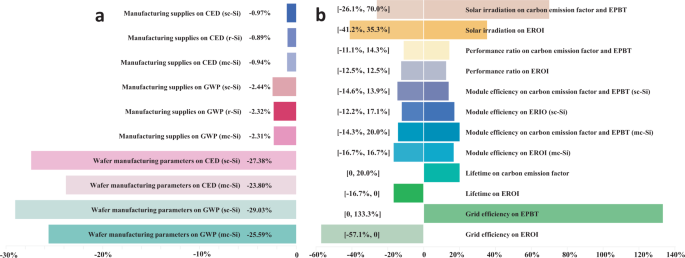
The sensitivity analysis includes: ( a ) manufacturing supply chain structure (number of representative trading partners) and wafer manufacturing parameters on cumulative energy demand (CED) and global warming potential (GWP), ( b ) solar irradiation, performance ratio, module efficiency, lifetime, and grid efficiency on energy payback time (EPBT), carbon emission factor, and energy return on investment (EROI).
We also perform sensitivity analysis of key parameters involved in the wafer manufacturing stage on energy and climate change impact. As an example of such an analysis, we show how reduced wafer thickness and kerf losses for slurry-based sawing would create an impact on CED and GWP when the wafers were manufactured in China in 2020. We find that the decline of these two parameters leads to a lessened amount of casted silicon and Czochralski silicon ingots needed for wafer production, which further leads to decreasing wafer weight input for PV cell and module production 44 . As a result, such changes in wafer thickness and kerf loss would cut CED by 27% (sc-Si) and 24% (mc-Si) and GWP by 29% (sc-Si) and 26% (mc-Si), as shown in Fig. 7a . On the other hand, according to the PV report by the Fraunhofer Institute for Solar Energy Systems that summarized the wafer thickness of c-Si PV cell development over the years 45 , the wafer thickness changed by no more than 5% from 2015 to 2020. Thus, we aim to mainly study and emphasize energy structure transitions and PV supplies instead, and the analysis regarding wafer thickness and kerf losses are considered in this sensitivity analysis.
We further investigate how variations in solar irradiation, performance ratio, module efficiency, lifetime, and grid efficiency can influence EPBT, carbon emission factor, and EROI. If grid efficiency increases from 30 to 70% 46 , 47 , EPBT can increase by 133%, and EROI can decrease by 57%, as shown in Fig. 7b . We see that through technological advancements, module efficiency is also anticipated to go up as a result of panel design improvements 6 , and we see an up to 17% increase in EROI and up to 15% decrease in carbon emission factor and EPBT as a result of improved module efficiency. We also take into consideration the degradation rates of c-Si PV panels which are reflected in their decreasing module efficiencies 48 . The results show that panel degradation leads to a 12% reduction in EROI for sc-Si and a 17% decrease for mc-Si, as well as a 14% increase in carbon emission factor and EPBT for sc-Si and a 20% increase for mc-Si. Another technological advancement we consider is the performance ratio 49 , which can lead to an 11% decrease in carbon emission factor if it reaches 90% and a 14% increase if it drops to 70%. Furthermore, we study the impacts of geographical location on these metrics from various levels of solar irradiation 47 . Regions with low solar irradiation come with higher carbon emission factors and EPBT, as shown by the 70% increase compared to medium irradiation regions, while regions with high solar irradiation demonstrate 26% lower carbon emission factor and EBPT. If the lifetime of the c-Si PV panel decreases by five years, the carbon emission factor would thus go up by 20%. The sensitivity analysis of various parameters can help us understand how technological advancements and geographical locations that place alongside manufacturing in the U.S. can have an impact on the energy and climate change metrics.
Reshoring c-Si PV manufacturing plays an important role in mitigating climate change. This study investigates the long-term implications of the current trend toward building a resilient and reliable reshored PV manufacturing supply. Departing from foreign supplies and instead bringing manufacturing back home will provide the PV industry with an alternative to fall back on when disruptions resurface. Reshored PV panel manufacturing is not only a strategy to protect domestic industry from supply bottlenecks but also aligns with the ambitious climate policy by substantially reducing carbon emissions.
In this study, the offshore manufacturing cases from 2010 to 2020 are considered as the U.S. previously relied on major PV panel imports from Asia 12 . The reshored manufacturing scenario in 2020 is studied unilaterally to examine whether the “Make it in America” strategy alone can support the climate agenda to realize decarbonization goals when compared with the offshore manufacturing case in the same year. More reshored manufacturing scenarios in 2025, 2030, 2035, 2040, 2045, and 2050 are forecasted to study how the reshored strategy and the climate policy interact in the next few centuries, given the disruptive nature that global politics could have on the PV supply chain.
Reshoring manufacturing reduces climate change impact from PV panel production by 23%, leading to tremendous benefits for the climate. Manufacturing and trade policies, significant financial support and incentives, as well as strategic actions focused on the workforce, will facilitate the rebuilding and continued operation of PV panel manufacturing facilities 11 . As the U.S. PV demand growth continues in the future, there may be opportunities for future domestic manufacturing expansion. If the reshored manufacturing can be achieved in 2035, a 30% climate change mitigation from manufacturing c-Si PV panels is expected. If the reshored manufacturing can be realized in 2050, a 33% mitigation of the climate change impact from panel production is projected. These reductions matter not only in the manufacturing stage but also in the overall scheme of the PV panel life cycle. The manufacturing stage of the c-Si PV life cycle is where the majority of GHG emissions occur 31 . Compared to the manufacturing stage, the operations life cycle stage of the solar PV system generates modest GHG emissions that are close to zero, due to the relatively low operational and maintenance requirements of PV systems 31 . A 1 m 2 PV panel emits 0.27 kg CO 2 eq GHG and demands 48 MJ of energy at its use stage 33 , 34 . Similarly, the amount of GHG emissions generated from the end-of-life treatment stage is minimal. A 1 m 2 PV panel emits 0.57 kg CO 2 eq GHG and demands 74 MJ of energy at its end-of-life treatment stage 33 , 34 . Putting them in the overall context of PV panel lifetime emissions and energy impact, the GHG emissions from the use stage contribute to less than 0.20% of PV lifetime emissions, and those from the end-of-life management stage contribute to less than 0.41%, as shown in Supplementary Fig. 4 under Supplementary Discussion 1 : Manufacturing vs operations and maintenance vs end-of-life treatment. The energy use from the use stage contributes to less than 2.0% of lifetime energy use, and that from the end-of-life management stage contributes to less than 3.0%. Since most carbon emissions occur in the upstream manufacturing process, and contributions of emissions from the use stage and the disposal stage are generally low, we conclude that the climate change mitigations from panel manufacturing as a result of reshoring are significant in the overall lifetime emissions.
Based on our quantitative analysis, reshoring aligns with the ambitious climate target. As solar is expected to make up 40% of U.S. power by 2035 and up to 70–80% by 2050, this can only be made possible by producing more PV panels 11 . Although there are various emerging PV technologies, no alternative PV technology can displace c-Si quickly enough to achieve power sector decarbonization by 2035 50 . Developing the U.S. c-Si PV domestic supply could mitigate challenges related to production disruption, compete with demand from other industries or countries, and maintain a robust U.S. domestic solar manufacturing leadership 51 , 52 . The Inflation Reduction Act encourages U.S. supply chains to span clean technologies, including solar technology, to create opportunities for small businesses and invest in American workers and the PV industry 53 . The legislation offers specific tax incentives for businesses that manufacture solar products domestically, and includes important requirements around domestic sourcing, such as the use of domestic panels in solar projects, as well as around prevailing wages and apprenticeships to ensure that good-paying jobs are offered to boost American manufacturing and competitiveness 20 . Policymakers have also stepped-up attempts to restart the American PV industry to renew efforts to bring manufacturing back. Such proposals draw on the momentum of a growing domestic movement in support of a “Green New Deal”, which has promised decent manufacturing jobs as a result of investments in low-carbon technologies and increasingly justified climate policies 54 . As of now, some policies explicitly aim at reshoring, such as tariffs put in place by past administrations, are still in effect, and a broad investigation into gaps in domestic supply chains has also been launched 54 .
Offshore manufacturing in the past does not always align with the climate target. Apart from the reshored manufacturing scenarios in the future that are assumption driven and formulated based on reasonable predictions, we also examine the past outsourced cases that relied on global supplies to interpret the impacts behind the ever-changing supply chain and manufacturing locations, as well as the power mix of trading partners from 2010 to 2020. Manufacturing c-Si PV panels from outsourced locations result in more GHG emissions in 2015 than in 2010. Moreover, as manufacturing locations shift from China, Germany, Japan, and Mexico in 2010 to South Korea, Malaysia, and Vietnam in 2015, the energy usage from panel production increases by up to 10% as well. As global dynamics shift quickly and more and more emerging supply crises demand our attention, it is difficult to determine an outsourced procurement strategy that not only complies with the ambitious national climate policies but also assures that geopolitical tensions would have no influence on it. Supply disruptions and bottlenecks can occur at any time to threaten the growth of renewable solar power and the PV industry, and the changing manufacturing deployment on account of policies and demand can increase GHG emissions. To stabilize supply and attain consistent carbon emission reduction, switching to leading-edge domestic manufacturing is an option to consider. Doing so will enable the pursuit of strategic objectives, particularly those in the energy, climate, and national security domains.
Manufacturing c-Si PV panels is attractive to pursue domestically as reshored production demonstrates many more benefits. The domestic production of solar products also aids in building broader coalitions and offers possible spillover benefits for climate policy. Outsourcing production to other countries over time is not a sustainable business model 55 . Offshoring can potentially result in job losses, wage reduction, and disruption of business innovation and productivity, which leads to policymakers proposing anti-offshoring or reshoring bills and policies that provide tax incentives for domestic production 56 . A reshored manufacturing base in solar PV may provide benefits such as more direct local employment and a more resilient energy supply system. Foreign manufacturers may be risky, impractical, or undesirable partners for public funds, whereas establishing a strong link between public funding of research and development and the domestic private sector has been identified as crucial to achieving climate goals, both by lowering the risks of scale-up and by granting access to markets 57 . The reshoring decision and climate agendas harmonize to ramp up climate actions, as carbon emission factors and EPBT of c-Si PV reshored manufacturing in the future reduce dramatically. If reshored manufacturing can be achieved in 2035, among 31% of the reduction in carbon emission factor from panel production, reshoring leads to a 23% of emission factor decrease while renewable penetration to the power grid contributes to 8%. On the other hand, among 14% of EPBT reductions from panel manufacturing, 4% of EPBT declines are attributed to reshoring, while the remaining 10% are the credits of renewable penetration. Renewable penetration and reshoring work together to create enormous energy and climate benefits. Renewable penetration to the power grid can only be made possible through more c-Si PV solar panel manufacturing and can only be achieved as the solar panel industry expands. As the U.S. achieves its energy transition goal by 2035 and 2050, the reshored panel manufacturing will benefit from large shares of renewables in the power grid by then in return. Besides energy and environmental strengths, the import costs of c-Si manufacturing inputs add 11% to the total expenditure, while a build-up in the domestic PV supply chain from “cradle-to-site” would dramatically reduce the cost 11 . Despite the minimized cost, c-Si PV manufacturing materials are mostly benign and available in very large quantities and have demonstrated long-term durability 11 . Besides, along with reshored manufacturing and increasing renewable power sources, technological innovations and breakthroughs can help achieve lower carbon emission factors and EPBT (15% by module efficiency advancement, 11% by performance ratio improvement) from PV panel production by 2050. Apart from withstanding supply crisis, reshored manufacturing is appealing to implement due to the numerous advantages listed above, which can be harmonized with technological advancements and renewable penetration to the power grid, and the conclusion of this study has important implications for other regions or industries to secure a reliable supply base.
Methodology overview
In our LCA to study c-Si PVs, we follow the existing approach of setting the functional unit as a 1 m 2 c-Si PV module over the “cradle-to-site” PV life cycle system boundary from quartz mining to PV module production to shipment of PV panels 58 . The study is conducted in the OpenLCA 1.10.3 software with data imported from ecoinvent version 3.7.1 (2020) database and OpenLCA LCIA methods version 2.0.5 34 . We also used Microsoft Excel version 2212 for data analysis. The LCA we conducted is spatially explicit with all geographically specific inventories, mainly including raw materials and energy. We discuss the various elements of our approach: (1) elements of LCA, including goal and scope definitions, life cycle stages, life cycle inventory, and life cycle impact assessment; (2) integration of the global industrial structure change; (3) energy structure and compositions of power mix; (4) parameters for EPBT and carbon emission factor calculations. In this study, we focus on PV modules ultimately shipped to or manufactured in the U.S. We investigate all scenarios and cases applying three types of c-Si based technologies toward 2050, namely sc-Si, r-Si, and mc-Si, as shown in Supplementary Fig. 8 under Supplementary Methods 1 : Life cycle inventory and life cycle stages.
System boundary and analysis scope
In this work, we aim to evaluate the “cradle-to-site” climate change and energy impacts of reshored c-Si PV panel manufacturing to assess if the act of bringing manufacturing back home aligns with the climate target. The system boundary of the life cycle of c-Si PVs consists of several stages, from raw material acquisition to solar module production. We include all major and minor manufacturing and construction materials, from wet wood chips in quartz mining to low-iron solar glass in module production, in the inventory. To enable a fair comparison of modules in different cases and scenarios regarding material inputs, energy consumption, and emissions, we define the system boundary to be from silica sand mining to panel manufacturing to shipping panels to the U.S. for all for consistency. In this study, the overarching functional unit, typically defined in terms of a unit quantity of product, is set to be 1 m 2 of the solar module according to the previous literature 47 , 59 , which is helpful to capture the changes in energy and environmental profiles proportional to PV size directly over time. We note that the end-of-life phase is excluded from the system boundary following assumptions in existing literature accounting for lack of data 7 , as there has been insufficient data on the disposal phase as well as the balance of plants 60 .
Life cycle stages
The manufacture of PV modules involves several stages, from quartz mining to PV module production, as shown in Fig. 2 . The system starts with silica sand acquisition, of which only heat and sand are added to the first stage to obtain silica sand 61 . Metallurgical grade silicon, a crucial stepping stone in the refining process of silicon metals, is then yielded by a carbothermic reduction reaction from silica sand with other material inputted, including petroleum coke, wet wood chips, etc., into the second stage 62 . After metallurgical grade silicon is obtained, electronics grade silicon is produced through the Siemens process, which involves the deposition of silicon from a mixture of purified silane with an excess of liquid hydrogen onto high purity metallurgical grade silicon. Solar grade silicon is produced through a modified Siemens process, which involves additional processing to separate the toxic and corrosive gas from the reduction process of metallurgical grade silicon 63 , 64 . These procedures to obtain all these types of silicons are homogeneous regardless of c-Si technology type, although the quantities needed to produce the same functional unit of three types of c-Si PV modules are different. After solar grade and electronics grade silicon are obtained, the manufacturing configurations of PV systems start to differ by the type of c-Si selected as the semiconductor material to form cells and modules. When sc-Si is the semiconductor material, the Czochralski crystal growth technique is implemented to form sc-Si crystal blocks in an inert atmosphere, such as argon in this study 65 . These crystals then go through the wafer sawing process in that individual silicon chips are mechanically separated from each other for cell manufacturing 66 . When r-Si is the semiconductor material, solar grade silicon and electronics grade silicon are used directly for r-Si wafer production, of which carbon-based strings are pulled upward through holes with molten silicon, and sawing loss is avoided 67 , leading to relatively low energy required to manufacture r-Si PV module compared with sc-Si and mc-Si technologies. When mc-Si is picked as the semiconductor material, solar grade silicon and electronics grade silicon are melted and cast into quartz crucibles to form mc-Si ingots 68 . Similar to sc-Si crystals, mc-Si ingots then go through the process of wafer sawing 69 . Processing of silicon wafers into solar cells involves texturing, acid cleaning, diffusion, etching, etc., while electrical contacts are placed between the cells and then wired and arrayed to form modules. Despite the differences in wafer types, the cell manufacturing and module assembly processes are similar for all three types of c-Si technologies 70 .
Life cycle inventory
The LCI in our study embraces mass and energy balance involved in each life cycle stage, integrating the trade structure of products as well as power mix inputs. We derive supply chain structure from the trade data for c-Si PV cells and general solar cells assembled into modules or panels 36 , and more details are discussed under Supply chain structure and information. The relevant LCI regarding energy information of global PV exporting regions in the past, as well as power mix predictions in the future are obtained, which are discussed in more detail under Energy structure 8 , 39 . General mass and energy balance data throughout the life cycle of PV are collected from ecoinvent version 3.7.1 and relevant literature 34 .
Life cycle impact assessment
Primary energy consumption and the intergovernmental panel on climate change (IPCC) 2013 method are selected as the primary LCIA methods in this study, as it is imperative to comprehend the energy and decarbonization implications of promising energy suppliers: PVs. Several prevailing sustainability metrics are calculated based on the CED and GWP, respectively. GWP was illustrated over an integrated time horizon of 100 years, using the impact assessment method described by IPCC 2013 GWP 100a 71 , 72 . We first normalize the life cycle GWP on a 1 m 2 PV module basis, then analyze the corresponding life cycle stage breakdown. EPBT, the time needed to generate as much energy as consumed during the production stages, is an essential metric adopted widely in characterizing the energy sustainability of PV technologies. EPBT is dominated by energy embedded in raw materials and energy consumed in manufacturing products. EROI, the amount of energy expended to produce a certain amount of energy, is another critical metric proportional to the inverse of EPBT. Besides metrics that describe the energy use, the carbon emission factor or life cycle carbon emission factor, the total amount of GHG emission mainly induced from material production and PV manufacturing is also a crucial metric describing the climate change impact of the PV system. When calculating these metrics, we account for the geographical and temporal influence on input parameters, including solar irradiation, module efficiency, etc. Additional life cycle impact assessment results are presented in Supplementary Discussion 4 : Life cycle impact assessment results.
Supply chain structure and information
The trade data are collected from USITC and calculated as market shares in each offshore manufacturing case, as shown in Fig. 5 and Table 1 36 . All trade information can be obtained by using the Harmonized Tariff Schedule (HTS) code, which is the primary tool applied for data search in this study. We also refer to the ruling references on tariff classification in customs rulings online search system (CROSS) from U.S. Customs and Border Protection regarding solar PV product HTS codes. Based on the ruling references provided in CROSS, trade data are obtained based on HTS code 8541.40.6015 representing c-Si PV cells of a thickness equal to or greater than 20 mm assembled into modules or panels, and more subdivisions on the type of c-Si are not available. The trade data corresponding to c-Si PV is only available for years after 2018. Since no data are available back in 2010 and 2015 using the former HTS code representing c-Si PV commodities, we select another HTS code 8541.40.6020, that represents solar cells assembled into modules or panels without the type of solar cells specified, which are retrievable in 2010 and 2015. The corresponding trade data are the best available for offshore manufacturing cases before 2018. The percentage distributions of solar PV supply market share for the U.S. are thus calculated from the trade data. Additional information regarding supply chain structure are provided in Supplementary Note 1 : Supply chain and trade data.
Energy structure
We obtain time series electricity generation by source data of each region from decades ago to recent years 39 . Typical electricity sources include coal, oil, natural gas, hydropower, nuclear, wind, etc. As the annual electricity generation increases for most regions over the years, the shares of sources also vary dramatically based on each region’s individual productivity advancements, energy demand requests, energy policy emphasis, and carbon neutrality targets. The data in 2010 and 2015 were retrieved directly, while the data used to represent the 2020 case are the closest to 2020, as shown in Supplementary Fig. 10 .
The energy structures of different trading partners as well as the corresponding emissions gerneated from each region’s unit power usage have differed drastically in the past ten years, as shown in Table 2 . While China’s coal demand and production capacity remained high, the government pushed to reduce emissions, improve air quality, and enhance the competitiveness of wind and solar PV in the electricity network. In Malaysia, the share of natural gas in the power mix continued to decrease by roughly 10% every five years, from 67% in 2005 to 57% in 2010, and from 47% in 2015 to 37% in 2019, responding to the policy of switching to coal and hydro fuels. Japan was one of the few countries with a decrease in power generation from 2010 to 2020 based on its energy security, economic efficiency, as well as environmental sustainability principles. Vietnam relied more on hydropower than other countries, but its coal-fired capacity was on the rise until 2019. South Korea’s energy sector was characterized by a predominance of fossil fuels, including coal, natural gas, and nuclear power, on par with Taiwan’s. Singapore, Thailand, and Mexico relied heavily on natural gas as their primary power source. In contrast, Germany relied on a wide variety of fuels, with a significant increase in the contribution of wind power from 2010 to 2020. While most regions in the world still rely on natural gas and coal as major sources of electricity, most have set goals to diversify the energy mix soon, phasing out traditional fossil fuels and increasing the share of renewables.
The U.S. energy policy landscape has also varied fundamentally over time in its ability to provide a renewable, affordable, and environmentally sustainable energy system to be anticipated in the future. The U.S. fuel mix for power generation has undergone a considerable shift, with coal power declining from around 20% to 13% and renewables rapidly growing from around 20% to 38%, driven by lower costs and policy support. Policies at the state and federal levels have encouraged significant investment in renewable energy generation. The fuel mix projection assumes no consideration of long-term structural changes in electricity demand accounting for the pandemic 8 . New capacity additions come primarily from natural gas and, increasingly, renewable technologies, as generating capacities of coal and nuclear retire. Incentives for renewables and declining technology costs support intense competition with natural gas. With the share of natural gas generating remaining relatively stable and the contribution of coal and nuclear halving, renewables will more than double their share from 2020 to 2050, as shown in Table 3 . Electricity demand will grow at a modest rate throughout the forecast period. The AEO projects that renewable generation will grow faster than overall electricity demand through 2050. Wind marks the main contributor to the growth in renewable generation before 2024, accounting for more than two-thirds of the growth over this period. After the production tax credit for wind energy were to be phased out at the end of 2024, solar power would account for almost three-quarters of the growth in renewable generation.
The grid mix efficiency indicates the average primary solar energy to electricity conversion efficiency at the demand side, usually between 6% and 40% for solar cells. In this paper, the grid mix efficiency is set to be 30% based on an average thermal to electrical energy conversion efficiency 47 , 73 . Another grid mix efficiency of 70% is selected as an upper bound of grid efficiency for sensitivity analysis 46 , 47 .
The annual electricity generation is calculated as the product of performance ratio, solar irradiation, and module efficiency used for calculating carbon emission factor and EPBT. When the actual specific yield is plotted against the rated annual irradiation in the module plane, the performance ratio marks the slope of the resulting regression line 45 , 47 . The typical performance ratio was around 50% to 80% in 1994 and 1997 with an average of 70% and large variance, and from 2010 onwards, it is typically around 70–90% with minor variance in performance ratio compared to 1990’s 45 . We apply the default performance ratio of 80% for a utility-scale PV system 44 , 74 . We also selected an upper bound of 90% and a lower bound of 70% of the performance ratio for the sensitivity analysis 45 , 49 . Performance ratio measures the quality of a PV plant to render the proportion of energy available for export to the grid after deduction of thermal losses, conduction losses, and energy consumed in operation, making it independent of location and often described as a quality factor 75 . Therefore, we assume that the performance ratio does not differ significantly between cases and scenarios. The solar irradiation level is assumed to be 1700 kWh/m 2 /year (medium level), a high level of 2300 kWh/m 2 /year, and a low level of 1000 kWh/m 2 /year are used for the sensitivity analysis. Total solar irradiance varies slowly on decadal and longer timescales, and variation during solar cycle 21 was about 0.1%, so it is assumed that the solar irradiance does not fluctuate significantly over time between different cases and scenarios 76 , 77 .
The conversion efficiencies of modules made of any of the three main types of silicon wafers have generally increased over time 78 , 79 . The efficiencies of average commercial wafer-based silicon modules increased from about 15% in 2010 to 20% in 2020, and record efficiencies demonstrate the potential for even further efficiency enhancements at the production level, although a physical limit for silicon solar cell conversion efficiency exists 45 . Over the past decade, mainstream module efficiency increased by 0.3–0.4% absolute per year on average 32 . We first assume a module efficiency of 14%, 11%, and 13% in 2010 for sc-Si, r-Si, and mc-Si technologies, respectively 33 , 70 . The sc-Si, r-Si, and mc-Si module efficiencies are assumed and estimated to be 17%, 13%, and 16% in 2015 6 , 47 . These numbers are further updated to 20.5%, 15%, and 18% in 2020 and onwards 6 , 47 . The efficiencies of modules sold in 2021 typically range from 17.4% (low-grade multi-crystalline cells) to 22.7% (high-performance back-contacted cells), with an estimated average of 20% for the most produced technology 32 . As the best cell efficiency so far for sc-Si is 26.7% and for mc-Si is 23.3% 80 , moving from individual wafers to full modules, there is a systematic difference between the module efficiency and the individual cell efficiency, and the cell-to-module efficiency ratio usually falls between 85% and 90% 32 . Therefore, in our sensitivity analysis, we assume the upper bound of the sc-Si module ratio to be 24%, that of r-Si to be 18%, and that of mc-Si to be 21%. Furthermore, we take into account degradation at a rate of 0.5% annually, which would result in a 2% reduction of module efficiency over 30 years 48 , and assumed the lower bound of sc-Si, r-Si, and mc-Si module efficiencies to be 18%, 13%, and 15% considering degradation in the sensitivity analysis.
Reporting summary
Further information on research design is available in the Nature Portfolio Reporting Summary linked to this article.
Data availability
All data needed to evaluate the conclusions in the paper are present in the main paper, the Supplementary Information, as well as https://github.com/PEESEgroup/c-Si-PV -. The LCIA data used in this study are available in the ecoinvent database [ https://ecoinvent.org/the-ecoinvent-database/ ] and the supply chain data used in this study are available from the United States International Trade Commission [ https://dataweb.usitc.gov/ ].
Graham F. COP26: Glasgow Climate Pact signed into history. Nat. Briefing https://doi.org/10.1038/d41586-021-03464-9 (2021).
Mercure, J.-F. et al. Reframing incentives for climate policy action. Nat. Energy 6 , 1133–1143 (2021).
Article ADS Google Scholar
Tavoni, M. et al. Post-2020 climate agreements in the major economies assessed in the light of global models. Nat. Clim. Change 5 , 119–126 (2015).
Rogelj, J. et al. Paris Agreement climate proposals need a boost to keep warming well below 2 C. Nature 534 , 631–639 (2016).
Article ADS CAS PubMed Google Scholar
Fact Sheet: President Biden Sets 2030 Greenhouse gas pollution reduction target aimed at creating good-paying union jobs and securing US leadership on clean energy technologies. (The White House, 2021).
Ballif, C., Haug, F.-J., Boccard, M., Verlinden, P. J. & Hahn, G. Status and perspectives of crystalline silicon photovoltaics in research and industry. Nat. Rev. Mater. 7 , 597–598 (2022).
Rabaia, M. K. H. et al. Environmental impacts of solar energy systems: A review. Sci. Total Environ. 754 , 141989 (2021).
Annual Energy Outlook 2021. (U.S. Energy Information Administration, U.S. Department of Energy, 2021).
Feldman, D. & Margolis, R. H2: Solar Industry Update. (NREL, 2021).(2020).
Tabassum, S. et al. Solar energy in the United States: development, challenges and future prospects. Energies 14 , 8142 (2021).
Article CAS Google Scholar
Paul, B. & David, F. Solar Photovoltaics Supply Chain Deep Dive Assessment. (U.S. Department of Energy, (2022).
Energy Information Administration 2020 Annual Solar Photovoltaic Module Shipment Report (2021).
Srivastava, H. Crystalline Silicon PV Market by Type (Mono-Crystalline and Multi-Crystalline) and End-User (Residential and Commercial, Utility-Scale)-Global Opportunity Analysis and Industry Forecasts, 2014–2022. (2016).
Woodhouse, M. et al. The role of advancements in solar photovoltaic efficiency, reliability, and costs. (NREL, 2016).
Strange, R. The 2020 Covid-19 pandemic and global value chains. J. Ind. Bus. Econ. 47 , 455–465 (2020).
Article Google Scholar
Iakovou, E. & White, C. How to build more secure, resilient, next-gen US supply chains. (2020).
Althaf, S. & Babbitt, C. W. Disruption risks to material supply chains in the electronics sector. Resour. Conserv. Recycling 167 , 105248 (2021).
Reshoring and the Pandemic: Bringing Manufacturing Back to America (2022).
Vaidyanathan, G. Scientists welcome’enormous’ US climate bill-but call for stronger action. Nature (2022).
Tollefson, J. How the biggest US energy bill ever could revive Biden’s climate agenda. Nature 608 , 458–459 (2022).
Theyel, G., Hofmann, K. & Gregory, M. Understanding manufacturing location decision making: rationales for retaining, offshoring, reshoring, and hybrid approaches. Econ. Dev. Q. 32 , 300–312 (2018).
Sayem, A., Feldmann, A. & Ortega-Mier, M. Investigating the influence of network-manufacturing capabilities to the phenomenon of reshoring: an insight from three case studies. BRQ Bus. Res. Q. 22 , 68–82 (2019).
Wang, M. et al. Breaking down barriers on PV trade will facilitate global carbon mitigation. Nat. Commun. 12 , 1–16 (2021).
Google Scholar
Goldthau, A. & Hughes, L. Nature Publishing Group Protect global supply chains for low-carbon technologies (2020).
Lin, J. et al. Carbon and health implications of trade restrictions. Nat. Commun. 10 , 1–12 (2019).
Lu, J., Mao, X., Wang, M., Liu, Z. & Song, P. Global and national environmental impacts of the US–China Trade War. Environ. Sci. Technol. 54 , 16108–16118 (2020).
Liu, L.-J., Creutzig, F., Yao, Y.-F., Wei, Y.-M. & Liang, Q.-M. Environmental and economic impacts of trade barriers: the example of China–US trade friction. Resour. Energy Econ. 59 , 101144 (2020).
Lu, J., Lemos, M. C., Koundinya, V. & Prokopy, L. S. Scaling up co-produced climate-driven decision support tools for agriculture. Nature Sustainability , https://doi.org/10.1038/s41893-021-00825-0 (2021).
Promises to keep. Nat. Clim. Change 12 , https://doi.org/10.1038/s41558-022-01480-9 .
Developing, U. Achieving American Leadership in the Solar Photovoltaics Supply Chain.
Hsu, D. D. et al. Life cycle greenhouse gas emissions of crystalline silicon photovoltaic electricity generation: systematic review and harmonization. J. Ind. Ecol. 16 , S122–S135 (2012).
Frankl, P., Menichetti, E., Raugei, M., Lombardelli, S. & Prennushi, G. Final report on technical data, costs and life cycle inventories of PV applications. Under Sixth Framework Programme, Project (2005).
Fthenakis, V. M. & Kim, H. C. Photovoltaics: Life-cycle analyses. Sol. Energy 85 , 1609–1628 (2011).
Article ADS CAS Google Scholar
Wernet, G. et al. The ecoinvent database version 3 (part I): overview and methodology. Int. J. Life Cycle Assess. 21 , 1218–1230 (2016).
Frischknecht, R., Wyss, F., Büsser Knöpfel, S., Lützkendorf, T. & Balouktsi, M.Cumulative energy demand in LCA: the energy harvested approach. Int. J. Life Cycle Assess. 20 , 957–969 (2015).
International Trade Commission Crystalline Silicon Photovoltaic Cells, Whether or Not Partially or Fully Assembled Into Other Products .(Accessed 10/30/2021) (2021).
Mineral Commodity Summaries. (U.S. Geological Survey, 2021) 2021.
Jäger-Waldau, A. PV status report 2019. (2019).
World Energy Outlook .(International Energy Agency, 2020) 2020.
Association, N. H. Washington, DC: FERC Facts you should know about hydropower (1999).
Davidson, D. J. Exnovating for a renewable energy transition. Nat. Energy 4 , 254–256 (2019).
Eroğlu, H. & Erdem, C. Solar energy sector under the influence of Covid-19 pandemic: A critical review. J. Energy Syst. 5 , 244–251 (2021).
Biden Jr, J. R. Executive Office of the President Washington DC Interim national security strategic guidance (2021).
Frischknecht, R. et al. Methodology Guidelines on Life Cycle Assessment of Photovoltaic 2020, IEA PVPS Task 12, IEA-PVPS. (2020).
Photovoltaics Report. (Fraunhofer Institute, Munich, Germany, 1−43 (2022).
Raugei, M., Peluso, A., Leccisi, E. & Fthenakis, V. Life-cycle carbon emissions and energy return on investment for 80% domestic renewable electricity with battery storage in California (USA). Energies 13 , 3934 (2020).
Fthenakis, V. & Leccisi, E. Updated sustainability status of crystalline silicon‐based photovoltaic systems: Life‐cycle energy and environmental impact reduction trends. Prog. Photovoltaics: Res. Appl. 29 , 1068–1077 (2021).
Bhandari, K. P., Collier, J. M., Ellingson, R. J. & Apul, D. S. Energy payback time (EPBT) and energy return on energy invested (EROI) of solar photovoltaic systems: A systematic review and meta-analysis. Renew. Sustain. Energy Rev. 47 , 133–141 (2015).
Reich, N. H. et al. Performance ratio revisited: is PR> 90% realistic? Prog. Photovoltaics: Res. Appl. 20 , 717–726 (2012).
Igogo, T. America’s Strategy to Secure the Supply Chain for a Robust Clean Energy Transition. (U.S. Department of Energy) (2022).
Smith, B., Woodhouse, M., Feldman, D. & Margolis, R. Solar Photovoltaic (PV) Manufacturing Expansions in the United States, 2017-2019: Motives, Challenges, Opportunities, and Policy Context. (2021).
Smith, B. & Margolis, R. M. Expanding the photovoltaic supply chain in the United States: opportunities and challenges. (2019).
Senate, U. Summary of the inflation reduction act of 2022 (2022).
Goldthau, A., Hughes, L. & Nahm, J. The Political Logic of Reshoring in Low Carbon Technologies: Economic Interdependence and Green Industrial Policy. Available at SSRN 4066047 (2022).
Whitfield, G. in Breaking up the Global Value Chain (Emerald Publishing Limited, 2017).
Sao, D. & Gupta, A. Threats to the international trade regime: Economic and legal challenges arising from anti-offshoring measures across the globe. Int’l Law 47 , 407 (2013).
Helveston, J. P., He, G. & Davidson, M. R. Quantifying the cost savings of global solar photovoltaic supply chains. Nature 1-5 (2022).
Muteri, V. et al. Review on life cycle assessment of solar photovoltaic panels. Energies 13 , 252 (2020).
Fthenakis, V., Kim, H. C., Held, M., Raugei, M. & Krones, J. Update of PV energy payback times and life-cycle greenhouse gas emissions. 24th European Photovoltaic Solar Energy Conference and Exhibition 21-25 (2009).
Fu, Y., Liu, X. & Yuan, Z. Life-cycle assessment of multi-crystalline photovoltaic (PV) systems in China. J. Clean. Prod. 86 , 180–190 (2015).
Darwis, D., Sesa, E., Kasim, S., Lestari, A. S. & Lamanu, M. Characteristic study of SiO2 content of quartz rock as a raw material for making silicon metal for solar cells. J. Phys.: Conf. Ser. 1434 , 012021 (2020).
CAS Google Scholar
Liu, Y. et al. Recycling high purity silicon from solar grade silicon cutting slurry waste by carbothermic reduction in the electric arc furnace. J. Clean. Prod. 224 , 709–718 (2019).
Chigondo, F. From metallurgical-grade to solar-grade silicon: an overview. Silicon 10 , 789–798 (2018).
Pizzini, S. Towards solar grade silicon: Challenges and benefits for low cost photovoltaics. Sol. Energy Mater. Sol. cells 94 , 1528–1533 (2010).
Vegad, M. & Bhatt, N. M. Review of some aspects of single crystal growth using Czochralski crystal growth technique. Procedia Technol. 14 , 438–446 (2014).
Goodrich, A. et al. A wafer-based monocrystalline silicon photovoltaics road map: Utilizing known technology improvement opportunities for further reductions in manufacturing costs. Sol. energy Mater. Sol. cells 114 , 110–135 (2013).
Reimann, C. et al. Systematic characterization of multi-crystalline silicon String Ribbon wafer. J. Cryst. growth 361 , 38–43 (2012).
Seigneur, H. et al. Manufacturing metrology for c-Si photovoltaic module reliability and durability, Part I: Feedstock, crystallization and wafering. Renew. Sustain. Energy Rev. 59 , 84–106 (2016).
Möller, H. J., Funke, C., Rinio, M. & Scholz, S. Multicrystalline silicon for solar cells. Thin Solid Films 487 , 179–187 (2005).
Yue, D., You, F. & Darling, S. B. Domestic and overseas manufacturing scenarios of silicon-based photovoltaics: Life cycle energy and environmental comparative analysis. Sol. Energy 105 , 669–678 (2014).
Forster, P. et al. Changes in atmospheric constituents and in radiative forcing . 39 (2007).
Pearce, W., Holmberg, K., Hellsten, I. & Nerlich, B. Climate change on Twitter: Topics, communities and conversations about the 2013 IPCC Working Group 1 report. PloS One 9 , e94785 (2014).
Article ADS PubMed PubMed Central Google Scholar
Leccisi, E., Raugei, M. & Fthenakis, V. The energy and environmental performance of ground-mounted photovoltaic systems—a timely update. Energies 9 , 622 (2016).
Syahindra, K. D., Ma’arif, S., Widayat, A. A., Fauzi, A. F. & Setiawan, E. A. Solar PV system performance ratio evaluation for electric vehicles charging stations in transit oriented development (TOD) areas. E3S Web Conf. 231 , 02002 (2021).
Khalid, A. M., Mitra, I., Warmuth, W. & Schacht, V. Performance ratio–Crucial parameter for grid connected PV plants. Renew. Sustain. Energy Rev. 65 , 1139–1158 (2016).
Kopp, G. Magnitudes and timescales of total solar irradiance variability. J. Space Weather Space Clim. 6 , A30 (2016).
Lean, J. L. Cycles and trends in solar irradiance and climate. Wiley Interdiscip. Rev. Clim. change 1 , 111–122 (2010).
Ludin, N. A. et al. Prospects of life cycle assessment of renewable energy from solar photovoltaic technologies: a review. Renew. Sustain. Energy Rev. 96 , 11–28 (2018).
Woodhouse, M. A., Smith, B., Ramdas, A. & Margolis, R. M. Crystalline silicon photovoltaic module manufacturing costs and sustainable pricing: 1H 2018 Benchmark and Cost Reduction Road Map. (NREL, 2019).
Green, M. et al. Solar cell efficiency tables (2021).
Commission, U. I. T. Interactive tariff and trade dataweb. accessed April 18, 1997–2003 (2022).
IEA. World energy balances. Energy Agency (2021).
EIA. World Energy Projection System. (2021).
Download references
Acknowledgements
This work is based upon work supported by the National Science Foundation (NSF) under Grant No. CBET-1643244 (to F.Y.).
Author information
Authors and affiliations.
Systems Engineering, Cornell University, Ithaca, NY, 14853, USA
Haoyue Liang & Fengqi You
Robert Frederick Smith School of Chemical and Biomolecular Engineering, Cornell University, Ithaca, NY, 14853, USA
Cornell Atkinson Center for Sustainability, Cornell University, Ithaca, NY, 14853, USA
You can also search for this author in PubMed Google Scholar
Contributions
F.Y. and H.L. contributed to the study design, data collection, analysis, and result interpretation. H.L. and F.Y. wrote the manuscript.
Corresponding author
Correspondence to Fengqi You .
Ethics declarations
Competing interests.
The authors declare no competing interests.
Peer review
Peer review information.
Nature Communications thanks the anonymous reviewer(s) for their contribution to the peer review of this work.
Additional information
Publisher’s note Springer Nature remains neutral with regard to jurisdictional claims in published maps and institutional affiliations.
Supplementary information
Supplementary information, reporting summary, rights and permissions.
Open Access This article is licensed under a Creative Commons Attribution 4.0 International License, which permits use, sharing, adaptation, distribution and reproduction in any medium or format, as long as you give appropriate credit to the original author(s) and the source, provide a link to the Creative Commons license, and indicate if changes were made. The images or other third party material in this article are included in the article’s Creative Commons license, unless indicated otherwise in a credit line to the material. If material is not included in the article’s Creative Commons license and your intended use is not permitted by statutory regulation or exceeds the permitted use, you will need to obtain permission directly from the copyright holder. To view a copy of this license, visit http://creativecommons.org/licenses/by/4.0/ .
Reprints and permissions
About this article
Cite this article.
Liang, H., You, F. Reshoring silicon photovoltaics manufacturing contributes to decarbonization and climate change mitigation. Nat Commun 14 , 1274 (2023). https://doi.org/10.1038/s41467-023-36827-z
Download citation
Received : 05 July 2022
Accepted : 16 February 2023
Published : 08 March 2023
DOI : https://doi.org/10.1038/s41467-023-36827-z
Share this article
Anyone you share the following link with will be able to read this content:
Sorry, a shareable link is not currently available for this article.
Provided by the Springer Nature SharedIt content-sharing initiative
This article is cited by
- Saeed Rahimpour Golroudbary
- Mari Lundström
- Benjamin P. Wilson
Scientific Reports (2024)
CO2 emissions of constructing China’s power grid towards carbon–neutral target: Based on top-down and bottom-up integrated model
Environmental Science and Pollution Research (2023)
By submitting a comment you agree to abide by our Terms and Community Guidelines . If you find something abusive or that does not comply with our terms or guidelines please flag it as inappropriate.
Quick links
- Explore articles by subject
- Guide to authors
- Editorial policies
Sign up for the Nature Briefing newsletter — what matters in science, free to your inbox daily.

Brad Parscale helped Trump win in 2016 using Facebook ads. Now he’s back, and an AI evangelist
Fort Lauderdale, Fla. — Donald Trump’s former campaign manager looked squarely into the camera and promised his viewers they were about to witness a bold new era in politics.
“You’re going to see some of the most amazing new technology in artificial intelligence that’s going to replace polling in the future across the country,” said Brad Parscale in a dimly lit promotional video accentuated by hypnotic beats.
Parscale, the digital campaign operative who helped engineer Trump’s 2016 presidential victory, vows that his new, AI-powered platform will dramatically overhaul not just polling, but campaigning. His AI-powered tools, he has boasted, will outperform big tech companies and usher in a wave of conservative victories worldwide.
It’s not the first time Parscale has proclaimed that new technologies will boost right-wing campaigns. He was the digital guru who teamed up with scandal-plagued Cambridge Analytica and helped propel Trump to the White House eight years ago. In 2020, he had a public blowup then a private falling out with his old boss after the Capitol riot. Now he’s back, playing an under-the-radar role to help Trump, the presumptive GOP nominee, in his race against Democratic President Joe Biden.
Parscale says his company, Campaign Nucleus, can use AI to help generate customized emails, parse oceans of data to gauge voter sentiment and find persuadable voters, then amplify the social media posts of “anti-woke” influencers, according to an Associated Press review of Parscale’s public statements, his company websites, slide decks, marketing materials and other documents not previously made public.
Since last year, Campaign Nucleus and other Parscale-linked companies have been paid more than $2.2 million by the Trump campaign, the Republican National Committee and their related political action and fundraising committees, campaign finance records show.
While his firms have received only a small piece of Trump’s total digital spending, Parscale remains close to top Republicans, as well as senior officials at the campaign and at the RNC, according to a GOP operative familiar with Parscale’s role who spoke on condition of anonymity to discuss internal dynamics.
Lara Trump, the RNC’s new co-chair and Trump’s daughter-in-law, once worked as a consultant to a company co-owned by Parscale. And U.S. House Speaker Mike Johnson's campaign recently hired Campaign Nucleus, campaign finance records show.
Parscale, however, is not involved in day-to-day Trump campaign operations, the GOP operative said.
Parscale’s ability to use AI to micro target supporters and tap them for campaign cash could prove critical for Trump’s campaign and other fundraising organizations. They have seen a falloff in contributions from smaller donors and a surge in spending — at least $77 million so far — on attorneys defending the former president in a slew of criminal and civil cases.
Beyond Trump, Parscale has said he’s harnessed AI to supercharge conservative candidates and causes across the globe, including in Israel, the Balkans and Brazil.
New AI-powered campaign tools
Parscale is hardly alone in using machine learning to try to give candidates an edge by predicting, pinpointing and motivating likely supporters to vote and donate money. Politicians at all levels are experimenting with chatbots and other generative AI tools to write speeches, ad copy and fundraising appeals.
Some Democrats have voiced concern over being outmaneuvered by Republicans on AI, much like they were on social media advertising eight years ago. So far, the Biden campaign and other Democrats said they are using AI to help them find and motivate voters and to better identify and defeat disinformation.
Election experts say they are concerned about AI’s potential to upend elections around the world through convincing deepfakes and other content that could mislead voters. Free and low-cost generative AI services have grown in sophistication, and officials worry they can be used to smear a candidate or steer voters to avoid the polls, eroding the public’s trust in what they see and hear.
Parscale has the financial backing to experiment to see what works in ways that other AI evangelists may not. That is thanks, in part, to his association with an evangelical Texas billionaire who is among the state’s most influential political donors.
Parscale did not respond to multiple messages from AP seeking comment. The RNC declined comment as well.
AI is 'so scary'
Trump has called artificial intelligence “ so scary " and "dangerous." His campaign, which has shied away from highlighting Parscale's role, said in an emailed statement that it did not “engage or utilize” tools supplied by any AI company.
“The campaign uses a set of proprietary algorithmic tools, like many other campaigns across the country, to help deliver emails more efficiently and prevent sign up lists from being populated by false information,” said campaign spokesman Steven Cheung.
While political consultants often hype their tactics to land new contracts, they can also be intensely secretive about the details of that work to avoid assisting rivals. That makes it difficult to precisely track how Parscale is deploying AI for the Trump campaign, or more broadly.
Parscale has said Campaign Nucleus can send voters customized emails and use data analytics to predict voters’ feelings. The platform can also amplify “anti-woke” influencers who have large followings on social media, according to his company’s documents and videos.
Parscale said his company also can use artificial intelligence to create “stunning web pages in seconds” that produce content that looks like a media outlet, according to a presentation he gave last month at a political conference, where he was not advertised in advance as a speaker.
“Empower your team to create their own news,” said another slide, according to the presentation viewed by AP.
Soon, Parscale says, his company will deploy an app that harnesses AI to assist campaigns in collecting absentee ballots in the same way DoorDash or Grubhub drivers pick up dinners from restaurants and deliver them to customers.
Chris Wilson, a Republican strategist who recently worked for a SuperPAC backing Florida Gov. Ron DeSantis’ failed presidential bid, said he has seen Campaign Nucleus’ platform and was “envious” of its capabilities and simplicity.
“Somebody could download Nucleus, start working with it and really begin to use it,” said Wilson.
Other political consultants, however, called Parscale’s AI-infused sales pitch largely a rehash of what campaigns already have mastered through data scraping, ad testing and modeling to predict voter behavior.
“Some of this stuff is just simply not new, it’s been around for a long time. The only thing new is that we’re just calling it AI,” said Amanda Elliott, a GOP digital strategist.
From unknown to Trump confidant
Parscale, a relatively unknown web designer in San Antonio, got his start working for Trump when he was hired to build a web presence for the business mogul’s family business.
That led to a job on the future president’s 2016 campaign. He was one of its first hires and spearheaded an ambitious and unorthodox digital initiative that relied on an extensive database of social media accounts and content to target voters with Facebook ads.
“I pretty much used Facebook to get Trump elected in 2016,” Parscale said in a 2022 podcast interview.
To better target Facebook users, in particular, the campaign teamed up with Cambridge Analytica, a British datamining firm bankrolled by Robert Mercer, a wealthy and influential GOP donor. After the election, Cambridge Analytica dissolved, facing investigations over its role in a breach of 87 million Facebook accounts.
Following Trump’s surprise win, Parscale’s influence grew. He was promoted to manage Trump's reelection bid and enjoyed celebrity status. A towering figure at 6 feet, 8 inches with a Viking-style beard, Parscale was frequently spotted at campaign rallies taking selfies with Trump supporters and signing autographs.
Parscale was replaced as campaign manager not long after a rally in Tulsa, Oklahoma, drew an unexpectedly small crowd, enraging Trump.
His personal life unraveled, culminating in a standoff with police at his Florida home after his wife reported he had multiple firearms and was threatening to hurt himself. One of the responding officers reported he saw bruising on the arms of Parscale’s wife. Parscale complied with a court order to turn in his firearms and was not charged in connection with the incident.
Parscale briefly decided to quit politics and privately expressed regret for associating with Trump after the Jan. 6, 2021, Capitol riot. In a text to a former campaign colleague, he wrote he felt “guilty for helping him win” in 2016, according to the House committee that investigated the Capitol attack.
His disgust didn’t last long. Campaign Nucleus set up Trump’s website after Silicon Valley tech companies throttled his access to their platforms.
By the summer of 2022, Parscale had resumed complimenting his old boss on a podcast popular among GOP politicos.
“With President Trump, he really was the guy driving the message. He was the chief strategist of his own political uprising and management,” Parscale said. “I think what the family recognized was: I had done everything that really the campaign needs to do.”
Parscale's platform
Trump’s 2024 campaign website now links directly to Parscale’s company and displays that it’s “Powered by Nucleus,” as Parscale often refers to his new firm. The campaign and its related political action and campaign committees have paid Campaign Nucleus more than $800,000 since early 2023, according to Federal Election Commission filings.
Two other companies — Dyspatchit Email and Text Services and BCVM Services — are listed on campaign finance records as being located at the same Florida address used by Campaign Nucleus. The firms, which are registered in Delaware and whose ownership is unclear, have received $1.4 million from the Trump campaign and related entities, FEC records show.
When an AP reporter last month visited Campaign Nucleus’ small, unmarked office in a tony section of Fort Lauderdale, an employee said she did not know anything about Dyspatchit or BCVM.
“We don’t talk to reporters,” the employee said.
The three companies have been paid to host websites, send emails, provide fundraising software and provide digital consulting, FEC records show.
Parscale markets Campaign Nucleus as a one-stop shop for conservative candidates who want to automate tasks usually done by campaign workers or volunteers.
The company says it has helped its clients raise $119 million and has sent nearly 14 billion emails on their behalf, according to a promotional video.
At his recent appearance at the political conference, Parscale presented a slide that said Campaign Nucleus had raised three times as much as tech giant Salesforce in head-to-head tests for email fundraising.
Campaign Nucleus specializes in mining information from a politician’s supporters, according to a recent presentation slide.
For example, when someone signs up to attend an event, Nucleus uses AI to analyze reams of personal data to assign that person a numerical score. Attendees who have been to past events receive a high score, for example, ranking them as most likely to show up, according to a company video posted online.
Campaign Nucleus also can track where people who sign up live and can send them customized emails asking for donations or solicit their help on the campaign, the video shows.
Parscale said two years ago in a podcast that he had received more than 10,000 requests about Campaign Nucleus from nearly every country with a conservative party. More recently, he said his team has been active in multiple countries, including in India and Israel, where he’s been “helping over there a lot with the war with Hamas.”
The company says it has offices in Texas, Florida and North Carolina and has been on a recruiting tear. Recent job listings have included U.S. and Latin America-based intelligence analysts to use AI for framing messages and generating content, as well as a marketer to “coordinate influencer campaigns.”
Campaign Nucleus has also entered into partnerships with other companies with an AI focus. In 2022, the firm announced it was teaming up with Phunware, a Texas-based company that built a cellphone app for Trump’s 2020 bid that allowed staff to monitor the movements of his millions of supporters and mobilize their social networks.
Since then, Phunware obtained a patent for what a company official described as “experiential AI” that can locate people’s cellphones geographically, predict their travel patterns and influence their consumer behavior.
Phunware did not answer specific questions about the partnership with Nucleus, saying the company's client engagements were confidential.
“However, it is well-known that we developed the 2020 Trump campaign app in collaboration with Campaign Nucleus. We have had discussions with Trump campaign leadership about potentially developing their app for the 2024 election," said spokeswoman Christina Lockwood.
Parscale's vision
Last year, Parscale bought property in Midland, Texas, in the heart of the nation’s highest-producing oil and gas fields. It is also the hometown of Tim Dunn, a billionaire born-again evangelical who is among the state’s most influential political donors.
Over the years, the organizations and campaigns Dunn has funded have pushed Texas politics further to the right and driven successful challenges to unseat incumbent Republican officials deemed too centrist.
In April 2023, Dunn invested $5 million in a company called AiAdvertising that once bought one of Parscale’s firms under a previous corporate name. The San Antonio-based ad firm also announced that Parscale was joining as a strategic adviser, to be paid $120,000 in stock and a monthly salary of $10,000.
“Boom!” Parscale tweeted. “(AiAdvertising) finally automated the full stack of technologies used in the 2016 election that changed the world.”
In June, AiAdvertising added two key national figures to its board: Texas investor Thomas Hicks Jr. – former co-chair of the RNC and longtime hunting buddy of Donald Trump Jr. -- and former GOP congressman Jim Renacci. In December, Dunn also gave $5 million to MAGA Inc., a pro-Trump super PAC and Campaign Nucleus client. And in January, SEC filings show Dunn provided AiAdvertising an additional $2.5 million via his investment company. A company press release said the cash infusion would help it “generate more engaging, higher-impact campaigns.”
Dunn declined to comment, although in an October episode of his podcast he elaborated on how his political work is driven by his faith.
“Jesus won’t be on the ballot, OK? Now, eventually, he’s going to take over the government and we can look forward to that,” Dunn told listeners. “In the meanwhile, we’re going to have to settle.”
In business filings, AiAdvertising said it has developed AI-created “personas” to determine what messages will resonate emotionally with its customers’ target audience. Parscale said last year in a promotional video that Campaign Nucleus was using AI models in a similar way.
“We actually understand what the American people want to hear,” Parscale said.
AiAdvertising did not respond to messages seeking comment.
Parscale occasionally offers glimpses of the AI future he envisions. Casting himself as an outsider to the Republican establishment, he has said he sees AI as a way to undercut elite Washington consultants, whom he described as political parasites.
In January, Parscale told a crowd assembled at a grassroots Christian event at a church in Pasadena, California, that their movement needed “to have our own AI, from creative large language models and creative imagery, we need to reach our own audiences with our own distribution, our own email systems, our own texting systems, our own ability to place TV ads, and lastly we need to have our own influencers.”
To make his point plain, he turned to a metaphor that relied on a decidedly 19th-century technology.
“We must not rely on any of their rails,” he said, referring to mainstream media and companies. “This is building our own train tracks.”
Burke reported from San Francisco. AP National Political Writer Steve Peoples and Courtney Subramanian in Washington, and Associated Press researcher Rhonda Shafner in New York contributed to this report.
This story is part of an Associated Press series, “The AI Campaign,” that explores the influence of artificial intelligence in the 2024 election cycle.
- Election 2024
- Entertainment
- Newsletters
- Photography
- Personal Finance
- AP Investigations
- AP Buyline Personal Finance
- AP Buyline Shopping
- Press Releases
- Israel-Hamas War
- Russia-Ukraine War
- Global elections
- Asia Pacific
- Latin America
- Middle East
- Election Results
- Delegate Tracker
- AP & Elections
- Auto Racing
- 2024 Paris Olympic Games
- Movie reviews
- Book reviews
- Personal finance
- Financial Markets
- Business Highlights
- Financial wellness
- Artificial Intelligence
- Social Media
Brad Parscale helped Trump win in 2016 using Facebook ads. Now he’s back, and an AI evangelist
FILE - People hold their cellphones as they wait for the plane carrying former President Donald Trump to take off after a campaign rally at Waco Regional Airport Saturday, March 25, 2023, in Waco, Texas. (AP Photo/Nathan Howard, File)
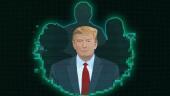
Brad Parscale was the digital campaign operative who helped engineer Trump’s 2016 presidential victory with the heavy use of Facebook ads. Now Parscale is back and is an evangelist for artificial intelligence. (AP animation: Eva Malek)
- Copy Link copied
FILE - Former President Donald Trump speaks during a commit to caucus rally, Friday, Jan. 5, 2024, in Mason City, Iowa. Political consultant Brad Parscale’s company, Campaign Nucleus, is boosting fundraising and voter engagement for the Trump campaign and other right-wing causes. Parscale says he leverages data science to automate how political operatives identify and motivate their supporters. (AP Photo/Charlie Neibergall, File)
FILE - Brad Parscale, then-campaign manager for President Donald Trump, speaks during a campaign rally at the Target Center in Minneapolis, Oct. 10, 2019. Parscale, the digital campaign operative who helped engineer Trump’s 2016 presidential victory, vows that his new, AI-powered platform will dramatically overhaul not just polling, but also campaigning. (AP Photo/Evan Vucci, File)
Pages from the Campaign Nucleus website are seen on a computer in New York on Thursday, May 2, 2024. Political consultant Brad Parscale’s company, Campaign Nucleus, is boosting fundraising and voter engagement for the Trump campaign and other right-wing causes by leveraging data science to automate how political operatives identify and motivate their supporters. (AP Photo/Patrick Sison)
FILE - Republican presidential candidate former President Donald Trump speaks at an election night rally on primary election night in Nashua, N.H., Tuesday, Jan. 23, 2024. Election experts say they are concerned about AI’s potential to upend elections around the world through convincing deepfakes and other content that could mislead voters. (AP Photo/David Goldman, File)
FILE - In this Tuesday, Oct. 15, 2019, file photo, Brad Parscale, then-campaign manager to President Donald Trump, speaks to supporters during a panel discussion, in San Antonio. Since 2023, Campaign Nucleus and other Parscale-linked companies have been paid about $2 million by the Trump campaign, the Republican National Committee and their related political action and fund-raising committees, according to campaign finance records. (AP Photo/Eric Gay, File)
People walk through a business park at 1512 Broward Blvd., in Fort Lauderdale, Fla., on Thursday, March 28, 2024, where Campaign Nucleus’s small, unmarked office is located. Political consultant Brad Parscale’s company is boosting fundraising and voter engagement for the Trump campaign. (AP Photo/Lynne Sladky)
FILE - An image of former President Donald Trump and Brad Parscale, former campaign manager for President Donald Trump, is displayed during a House select committee hearing investigating the Jan. 6 attack on the U.S. Capitol, on Capitol Hill in Washington, Thursday, Oct. 13, 2022. In a text to a former campaign colleague, Parscale wrote he felt “guilty for helping” Trump win after the Jan. 6 riots. (AP Photo/Andrew Harnik, Pool, File)
FILE - A supporter takes a photo as Republican presidential candidate former President Donald Trump speaks at a campaign rally Saturday, March 9, 2024, in Rome Ga. Phunware, a Texas-based company, built a cellphone app for Trump’s 2020 bid that allowed staff to monitor the movements of his millions of supporters and mobilize their social networks. Since then, Phunware obtained a patent for what a former company official described as “experiential AI” that can locate people’s cell phones geographically, predict their travel patterns and incentivize their consumer behavior. (AP Photo/Mike Stewart, File)
A warning sign is posted on a gate in a business park at 1512 Broward Blvd., in Fort Lauderdale, Fla., on Thursday, March 28, 2024, where Campaign Nucleus’s small, unmarked office is located. Political consultant Brad Parscale’s company is boosting fundraising and voter engagement for the Trump campaign and other right-wing causes. The company says it uses artificial intelligence to automate how political operatives identify and motivate their supporters, and to predict when and where they’re most likely to vote and make campaign donations. (AP Photo/Lynne Sladky)
FORT LAUDERDALE, Fla. (AP) — Donald Trump’s former campaign manager looked squarely into the camera and promised his viewers they were about to witness a bold new era in politics.
“You’re going to see some of the most amazing new technology in artificial intelligence that’s going to replace polling in the future across the country,” said Brad Parscale in a dimly lit promotional video accentuated by hypnotic beats.
Parscale, the digital campaign operative who helped engineer Trump’s 2016 presidential victory, vows that his new, AI-powered platform will dramatically overhaul not just polling, but campaigning. His AI-powered tools, he has boasted, will outperform big tech companies and usher in a wave of conservative victories worldwide.
It’s not the first time Parscale has proclaimed that new technologies will boost right-wing campaigns. He was the digital guru who teamed up with scandal-plagued Cambridge Analytica and helped propel Trump to the White House eight years ago. In 2020, he had a public blowup then a private falling out with his old boss after the Capitol riot. Now he’s back, playing an under-the-radar role to help Trump, the presumptive GOP nominee, in his race against Democratic President Joe Biden.
Parscale says his company Campaign Nucleus can use AI to help generate customized emails, parse oceans of data to gauge voter sentiment and find persuadable voters, then amplify the social media posts of “anti-woke” influencers, according to an Associated Press review of Parscale’s public statements, his company websites, slide decks, marketing materials and other documents not previously made public.
Experts warn AI deepfakes will aim to mislead voters and undermine elections around the world Here’s how governments and organizations are responding to the threat.
- In the U.S., the FCC outlawed robocalls containing AI-generated voices , which have been used to discourage voters.
- Major tech companies have signed an accord to prevent AI from being used to disrupt democratic elections worldwide.
- And a Davos report found AI-powered misinformation is the world’s biggest short-term threat .
AI-powered misinformation and disinformation are emerging risks as people in a slew of countries head to the polls. Read more on the 25 elections in 2024 that could change the world , and take a look at more of the AP’s global elections coverage.
Since last year, Campaign Nucleus and other Parscale-linked companies have been paid more than $2.2 million by the Trump campaign, the Republican National Committee and their related political action and fundraising committees, campaign finance records show.
While his firms have received only a small piece of Trump’s total digital spending, Parscale remains close to top Republicans, as well as senior officials at the campaign and at the RNC, according to a GOP operative familiar with Parscale’s role who spoke on condition of anonymity to discuss internal dynamics.
Lara Trump, the RNC’s new co-chair and Trump’s daughter-in-law, once worked as a consultant to a company co-owned by Parscale. And U.S. House Speaker Mike Johnson’s campaign recently hired Campaign Nucleus, campaign finance records show.
Parscale, however, is not involved in day-to-day Trump campaign operations, the GOP operative said.
FILE - In this Tuesday, Oct. 15, 2019, file photo, Brad Parscale, then-campaign manager to President Donald Trump, speaks to supporters during a panel discussion, in San Antonio. (AP Photo/Eric Gay, File)
Parscale’s ability to use AI to micro target supporters and tap them for campaign cash could prove critical for Trump’s campaign and other fundraising organizations. They have seen a falloff in contributions from smaller donors and a surge in spending — at least $77 million so far — on attorneys defending the former president in a slew of criminal and civil cases .
Beyond Trump, Parscale has said he’s harnessed AI to supercharge conservative candidates and causes across the globe, including in Israel, the Balkans and Brazil.
NEW AI-POWERED CAMPAIGN TOOLS
Parscale is hardly alone in using machine learning to try to give candidates an edge by predicting, pinpointing and motivating likely supporters to vote and donate money. Politicians at all levels are experimenting with chatbots and other generative AI tools to write speeches, ad copy and fundraising appeals.
Some Democrats have voiced concern over being outmaneuvered by Republicans on AI, much like they were on social media advertising eight years ago. So far, the Biden campaign and other Democrats said they are using AI to help them find and motivate voters and to better identify and defeat disinformation.
Election experts say they are concerned about AI’s potential to upend elections around the world through convincing deepfakes and other content that could mislead voters. Free and low-cost generative AI services have grown in sophistication, and officials worry they can be used to smear a candidate or steer voters to avoid the polls, eroding the public’s trust in what they see and hear.

This story is part of an Associated Press series, “The AI Campaign,” that explores the influence of artificial intelligence in the 2024 election cycle.
Parscale has the financial backing to experiment to see what works in ways that other AI evangelists may not. That is thanks, in part, to his association with a Texas billionaire who is among the state’s most influential political donors.
Parscale did not respond to multiple messages from AP seeking comment. The RNC declined comment as well.
AI IS ‘SO SCARY’
Trump has called artificial intelligence “ so scary " and “dangerous.” His campaign, which has shied away from highlighting Parscale’s role, said in an emailed statement that it did not “engage or utilize” tools supplied by any AI company.
“The campaign uses a set of proprietary algorithmic tools, like many other campaigns across the country, to help deliver emails more efficiently and prevent sign-up lists from being populated by false information,” said campaign spokesman Steven Cheung.
While political consultants often hype their tactics to land new contracts, they can also be intensely secretive about the details of that work to avoid assisting rivals. That makes it difficult to precisely track how Parscale is deploying AI for the Trump campaign, or more broadly.
Parscale has said Campaign Nucleus can send voters customized emails and use data analytics to predict voters’ feelings. The platform can also amplify “anti-woke” influencers who have large followings on social media, according to his company’s documents and videos.
Parscale said his company also can use artificial intelligence to create “stunning web pages in seconds” that produce content that looks like a media outlet, according to a presentation he gave last month at a political conference, where he was not advertised in advance as a speaker.
“Empower your team to create their own news,” said another slide, according to the presentation viewed by AP.
Pages from the Campaign Nucleus website are seen on a computer in New York on Thursday, May 2, 2024. (AP Photo/Patrick Sison)
Soon, Parscale says, his company will deploy an app that harnesses AI to assist campaigns in collecting absentee ballots in the same way DoorDash or Grubhub drivers pick up dinners from restaurants and deliver them to customers.
Chris Wilson, a Republican strategist who recently worked for a SuperPAC backing Florida Gov. Ron DeSantis’ failed presidential bid, said he has seen Campaign Nucleus’ platform and was “envious” of its capabilities and simplicity.
“Somebody could download Nucleus, start working with it and really begin to use it,” said Wilson.
Other political consultants, however, called Parscale’s AI-infused sales pitch largely a rehash of what campaigns already have mastered through data scraping, ad testing and modeling to predict voter behavior.
“Some of this stuff is just simply not new, it’s been around for a long time. The only thing new is that we’re just calling it AI,” said Amanda Elliott, a GOP digital strategist.
FROM UNKNOWN TO TRUMP CONFIDANT
Parscale, a relatively unknown web designer in San Antonio, got his start working for Trump when he was hired to build a web presence for the business mogul’s family business.
That led to a job on the future president’s 2016 campaign. He was one of its first hires and spearheaded an ambitious and unorthodox digital initiative that relied on an extensive database of social media accounts and content to target voters with Facebook ads.
“I pretty much used Facebook to get Trump elected in 2016,” Parscale said in a 2022 podcast interview.
To better target Facebook users, in particular, the campaign teamed up with Cambridge Analytica, a British datamining firm bankrolled by Robert Mercer, a wealthy and influential GOP donor. After the election, Cambridge Analytica dissolved, facing investigations over its role in a breach of 87 million Facebook accounts.
Following Trump’s surprise win, Parscale’s influence grew. He was promoted to manage Trump’s reelection bid and enjoyed celebrity status. A towering figure at 6 feet, 8 inches with a Viking-style beard, Parscale was frequently spotted at campaign rallies taking selfies with Trump supporters and signing autographs.
Parscale was replaced as campaign manager not long after a rally in Tulsa, Oklahoma, drew an unexpectedly small crowd, enraging Trump.
His personal life unraveled, culminating in a standoff with police at his Florida home after his wife reported he had multiple firearms and was threatening to hurt himself. One of the responding officers reported he saw bruising on the arms of Parscale’s wife. Parscale complied with a court order to turn in his firearms and was not charged in connection with the incident.
Parscale briefly decided to quit politics and privately expressed regret for associating with Trump after the Jan. 6, 2021, Capitol riot. In a text to a former campaign colleague, he wrote he felt “guilty for helping him win” in 2016, according to the House committee that investigated the Capitol attack.
FILE - An image of former President Donald Trump and Brad Parscale, former campaign manager for President Donald Trump, is displayed during a House select committee hearing investigating the Jan. 6 attack on the U.S. Capitol, on Capitol Hill in Washington, Thursday, Oct. 13, 2022. (AP Photo/Andrew Harnik, Pool, File)
His disgust didn’t last long. Campaign Nucleus set up Trump’s website after Silicon Valley tech companies throttled his access to their platforms.
By the summer of 2022, Parscale had resumed complimenting his old boss on a podcast popular among GOP politicos.
“With President Trump, he really was the guy driving the message. He was the chief strategist of his own political uprising and management,” Parscale said. “I think what the family recognized was: I had done everything that really the campaign needs to do.”
PARSCALE’S PLATFORM
Trump’s 2024 campaign website now links directly to Parscale’s company and displays that it’s “Powered by Nucleus,” as Parscale often refers to his new firm. The campaign and its related political action and campaign committees have paid Campaign Nucleus more than $800,000 since early 2023, according to Federal Election Commission filings.
Two other companies — Dyspatchit Email and Text Services and BCVM Services — are listed on campaign finance records as being located at the same Florida address used by Campaign Nucleus. The firms, which are registered in Delaware and whose ownership is unclear, have received $1.4 million from the Trump campaign and related entities, FEC records show.
When an AP reporter last month visited Campaign Nucleus’ small, unmarked office in a tony section of Fort Lauderdale, an employee said she did not know anything about Dyspatchit or BCVM.
“We don’t talk to reporters,” the employee said.
A warning sign is posted on a gate in a business park at 1512 Broward Blvd., in Fort Lauderdale, Fla., on Thursday, March 28, 2024, where Campaign Nucleus’s small, unmarked office is located. (AP Photo/Lynne Sladky)
The three companies have been paid to host websites, send emails, provide fundraising software and provide digital consulting, FEC records show.
Parscale markets Campaign Nucleus as a one-stop shop for conservative candidates who want to automate tasks usually done by campaign workers or volunteers.
The company says it has helped its clients raise $119 million and has sent nearly 14 billion emails on their behalf, according to a promotional video.
At his recent appearance at the political conference, Parscale presented a slide that said Campaign Nucleus had raised three times as much as tech giant Salesforce in head-to-head tests for email fundraising.
Campaign Nucleus specializes in mining information from a politician’s supporters, according to a recent presentation slide.
For example, when someone signs up to attend an event, Nucleus uses AI to analyze reams of personal data to assign that person a numerical score. Attendees who have been to past events receive a high score, for example, ranking them as most likely to show up, according to a company video posted online.
Campaign Nucleus also can track where people who sign up live and can send them customized emails asking for donations or solicit their help on the campaign, the video shows.
Parscale said two years ago in a podcast that he had received more than 10,000 requests about Campaign Nucleus from nearly every country with a conservative party. More recently, he said his team has been active in multiple countries, including in India and Israel, where he’s been “helping over there a lot with the war with Hamas.”
The company says it has offices in Texas, Florida and North Carolina and has been on a recruiting tear. Recent job listings have included U.S. and Latin America-based intelligence analysts to use AI for framing messages and generating content, as well as a marketer to “coordinate influencer campaigns.”
Campaign Nucleus has also entered into partnerships with other companies with an AI focus. In 2022, the firm announced it was teaming up with Phunware, a Texas-based company that built a cellphone app for Trump’s 2020 bid that allowed staff to monitor the movements of his millions of supporters and mobilize their social networks.
Since then, Phunware obtained a patent for what a company official described as “experiential AI” that can locate people’s cellphones geographically, predict their travel patterns and influence their consumer behavior.
Phunware did not answer specific questions about the partnership with Nucleus, saying the company’s client engagements were confidential.
“However, it is well-known that we developed the 2020 Trump campaign app in collaboration with Campaign Nucleus. We have had discussions with Trump campaign leadership about potentially developing their app for the 2024 election,” said spokeswoman Christina Lockwood.
PARSCALE’S VISION
Last year, Parscale bought property in Midland, Texas, in the heart of the nation’s highest-producing oil and gas fields. It is also the hometown of Tim Dunn, a billionaire born-again evangelical who is among the state’s most influential political donors.
Over the years, the organizations and campaigns Dunn has funded have pushed Texas politics further to the right and driven successful challenges to unseat incumbent Republican officials deemed too centrist.
In April 2023, Dunn invested $5 million in a company called AiAdvertising that once bought one of Parscale’s firms under a previous corporate name. The San Antonio-based ad firm also announced that Parscale was joining as a strategic adviser, to be paid $120,000 in stock and a monthly salary of $10,000.
“Boom!” Parscale tweeted. “(AiAdvertising) finally automated the full stack of technologies used in the 2016 election that changed the world.”
In June, AiAdvertising added two key national figures to its board: Texas investor Thomas Hicks Jr. – former co-chair of the RNC and longtime hunting buddy of Donald Trump Jr. -- and former GOP congressman Jim Renacci. In December, Dunn also gave $5 million to MAGA Inc., a pro-Trump super PAC and Campaign Nucleus client. And in January, SEC filings show Dunn provided AiAdvertising an additional $2.5 million via his investment company. A company press release said the cash infusion would help it “generate more engaging, higher-impact campaigns.”
Dunn declined to comment, although in an October episode of his podcast he elaborated on how his political work is driven by his faith.
“Jesus won’t be on the ballot, OK? Now, eventually, he’s going to take over the government and we can look forward to that,” Dunn told listeners. “In the meanwhile, we’re going to have to settle.”
FILE - Former President Donald Trump speaks during a commit to caucus rally, Friday, Jan. 5, 2024, in Mason City, Iowa. (AP Photo/Charlie Neibergall, File)
In business filings, AiAdvertising said it has developed AI-created “personas” to determine what messages will resonate emotionally with its customers’ target audience. Parscale said last year in a promotional video that Campaign Nucleus was using AI models in a similar way.
“We actually understand what the American people want to hear,” Parscale said.
AiAdvertising did not respond to messages seeking comment.
Parscale occasionally offers glimpses of the AI future he envisions. Casting himself as an outsider to the Republican establishment, he has said he sees AI as a way to undercut elite Washington consultants, whom he described as political parasites.
In January, Parscale told a crowd assembled at a grassroots Christian event at a church in Pasadena, California, that their movement needed “to have our own AI, from creative large language models and creative imagery, we need to reach our own audiences with our own distribution, our own email systems, our own texting systems, our own ability to place TV ads, and lastly we need to have our own influencers.”
To make his point plain, he turned to a metaphor that relied on a decidedly 19th-century technology.
“We must not rely on any of their rails,” he said, referring to mainstream media and companies. “This is building our own train tracks.”
Burke reported from San Francisco. AP National Political Writer Steve Peoples and Courtney Subramanian in Washington, and Associated Press researcher Rhonda Shafner in New York contributed to this report.
Contact AP’s global investigative team at [email protected] or https://www.ap.org/tips/
The Associated Press receives financial assistance from the Omidyar Network to support coverage of artificial intelligence and its impact on society. AP is solely responsible for all content. Find AP’s standards for working with philanthropies, a list of supporters and funded coverage areas at AP.org
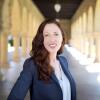
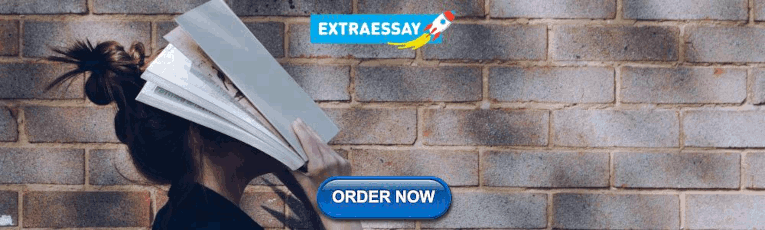
IMAGES
VIDEO
COMMENTS
1. Introduction. Silicon (Si), the second most abundant (~27%) element in the earth's crust after oxygen (~47%), is mostly present in the form of insoluble silicates such as magnesium silicate, aluminium silicate, iron silicate, calcium silicate, sodium, and potassium silicate (Bist et al., 2020).Besides several other forms of Si such as kaolin, smectite, vermiculite, quartz, amorphous silica ...
Silicon is one of the most important elements in the current age of the anthropocene. It has numerous industrial applications, and supports a high-tech multi-billion Euro industry. Silicon has a fascinating biological and geological cycle, interacting with other globally important biogeochemical cycles. In this review, we bring together both biological and geological aspects of the silicon ...
Silicon (Si) is the most abundant element on the earth's surface after oxygen. Si can be found in minerals, electronic chips, cosmetic products, and plants for instance. Here we review the Earth's terrestrial Si cycle, focussing on ecosystem services. We show that silicon can participate in the inherent and manageable properties involved in all ecosystem services. As records of the past ...
The global cycle of silicon has changed significantly over geological time from an early control by purely geochemical processes to control by biological processes, as occurs now (Siever, 1991). The concentration of DSi in the modern ocean is very low, ranging from less than 1 μM in open ocean surface waters to hundreds of μM in bottom waters.
The silica cycle is the biogeochemical cycle in which biogenic silica is transported between the Earth's systems. Silicon is considered a bioessential element and is one of the most abundant elements on Earth. The silica cycle has significant overlap with the carbon cycle (see carbonate-silicate cycle) and plays an important role in the sequestration of carbon through continental weathering ...
The preservation of sponge spicules in sediments was 45.2 ± 27.4%, but only 6.8 ± 10.1% for radiolarian testa and 8% for diatom frustules. Sponges lead to a global burial flux of 1.71 ± 1.61 ...
The element silicon (Si) is required for the growth of silicified organisms in marine environments, such as diatoms.These organisms consume vast amounts of Si together with N, P, and C, connecting the biogeochemical cycles of these elements.Thus, understanding the Si cycle in the ocean is critical for understanding wider issues such as carbon sequestration by the ocean's biological pump.
A new class of transporters are involved in the uptake of silicon from the environment into a diatom cell, with different levels of expression during the cell wall synthesis Hildebrand et al., 1997, Martin-Jézéquel et al., submitted.A relatively large number of studies have examined the kinetics of Si uptake by both natural diatom assemblages (Fig. 2) and cultured clones (see below).
Constraining the continental silicon cycle is a key requirement in attempts to understand both nutrient fluxes to the ocean and linkages between silicon and carbon cycling over different time scales. Silicon isotope data of dissolved silica (δ 30 Si DSi) are presented here from Lake Baikal and its catchment in central Siberia. As well as being ...
hypothesize that the modern ocean Si cycle is at approxi-mately steady state with inputs D14:8.2:6/Tmol Siyr1 and outputs D15:6.2:4/Tmol Siyr1. Potential impacts of global change on the marine Si cycle are discussed. 1 Introduction Silicon, the seventh most abundant element in the universe, is the second most abundant element in the Earth's ...
Silicon (Si) plays a crucial role in global biogeochemical cycles because it is an essential nutrient for a number of marine organisms, including some species of sponges, radiolarians ...
The soil there gave the researchers a look at the silicon cycle going back 2 million years. In analyzing soil samples from multiple depths, the researchers were able to compare old and ...
According to Roskill, in 2019, rechargeable batteries that are used in most green technologies, accounted for 54% of total lithium demand (Roskill, 2020). Statista reports that an estimated total of 82,000 metric tons of lithium was produced globally in 2020 while ten years earlier production was just 28,000 metric tons (Garside, 2021).
Silicon (Si) speciation and availability in soils is highly important for ecosystem functioning, because Si is a beneficial element for plant growth. Si chemistry is highly complex compared to other elements in soils, because Si reaction rates are relatively slow and dependent on Si species. Consequently, we review the occurrence of different Si species in soil solution and their changes by ...
1. Introduction [2] In a recent modeling study of the relative roles of nitrogen and phosphorus in controlling phytoplankton abundance, Tyrrell found that competition between algal groups that differed in their source of nitrogen for metabolism (NO 3 users versus N 2 fixers) was able to produce a "thermostat-like" effect which controlled the level of nitrogen in the surface ocean.
Silica is deposited extra- and intracellularly in plants in solid form, as phytoliths. Phytoliths have emerged as accepted taxonomic tools and proxies for reconstructing ancient flora, agricultural economies, environment, and climate. The discovery of silicon transporter genes has aided in the understanding of the mechanism of silicon transport and deposition within the plant body and ...
1.0 INTRODUCTION The mining sector will play a key role in the transition toward a low-carbon future. The technologies required to facilitate this shift, including wind turbines, solar panels and improved energy storage, all require significant mineral and metal inputs, and, absent any dramatic technological advances or an increase in the use of recycled materials, these inputs will come from ...
The life-cycle-focused sustainable mining framework can be summarized in the following way: the sustainability of mining and minerals consumption depends upon responsible rates of extraction and consumption of a mineral throughout its life cycle as well as the preservation of reserves and minimization of any losses. The life-cycle-focused ...
The evolution of the global carbon and silicon cycles is thought to have contributed to the long-term stability of Earth's climate 1-3.Many questions remain, however, regarding the feedback mechanisms at play, and there are limited quantitative constraints on the sources and sinks of these elements in Earth's surface environments 4-12.Here we argue that the lithium-isotope record can ...
Despite the growing concern about the importance of silicon (Si) in controlling ecological processes in aquatic ecosystems, little is known about its processing in riparian vegetation, especially compared to nitrogen (N) and phosphorus (P). We present experimental evidence that relative plant uptake of N and P compared to Si in riparian vegetation is dependent on mowing practices, water ...
Introduction. Mining is an economic activity capable of supporting the developmental goals of countries and societies. It also ensures that different metals, petroleum, and coal are available to different consumers or companies. Unfortunately, this practice entails excavation or substantial interference of the natural environment.
Reshoring silicon photovoltaic manufacturing back to the U.S. improves domestic competitiveness, advances decarbonization goals, and contributes to mitigating climate change.
Chemical equation: Si + 3HCl + 50 kWh/kg-Si 3 + H 2 50 Monocrystalline Silicon Monocrystalline silicon can be produced by further processing the EGS or UMGS in the Czochralski or float-zone pulling process. 15-20 Fractional Distillation The chlorsilanes are separated and high purity trichlorsilane SiHCl 3 gases are the product. 100 ...
Since last year, Campaign Nucleus and other Parscale-linked companies have been paid more than $2.2 million by the Trump campaign, the Republican National Committee and their related political ...
Campaign Nucleus set up Trump's website after Silicon Valley tech companies throttled his ... Campaign Nucleus specializes in mining information from a politician's supporters, according to a recent presentation slide. ... "The AI Campaign," that explores the influence of artificial intelligence in the 2024 election cycle. —-Contact ...