- Alzheimer's disease & dementia
- Arthritis & Rheumatism
- Attention deficit disorders
- Autism spectrum disorders
- Biomedical technology
- Diseases, Conditions, Syndromes
- Endocrinology & Metabolism
- Gastroenterology
- Gerontology & Geriatrics
- Health informatics
- Inflammatory disorders
- Medical economics
- Medical research
- Medications
- Neuroscience
- Obstetrics & gynaecology
- Oncology & Cancer
- Ophthalmology
- Overweight & Obesity
- Parkinson's & Movement disorders
- Psychology & Psychiatry
- Radiology & Imaging
- Sleep disorders
- Sports medicine & Kinesiology
- Vaccination
- Breast cancer
- Cardiovascular disease
- Chronic obstructive pulmonary disease
- Colon cancer
- Coronary artery disease
- Heart attack
- Heart disease
- High blood pressure
- Kidney disease
- Lung cancer
- Multiple sclerosis
- Myocardial infarction
- Ovarian cancer
- Post traumatic stress disorder
- Rheumatoid arthritis
- Schizophrenia
- Skin cancer
- Type 2 diabetes
- Full List »
share this!
September 9, 2021
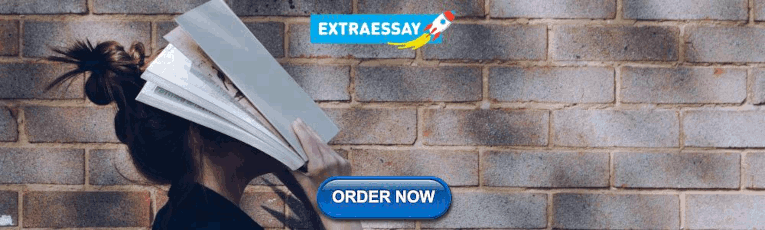
How the brain solves problems
by Delia Du Toit, Wits University
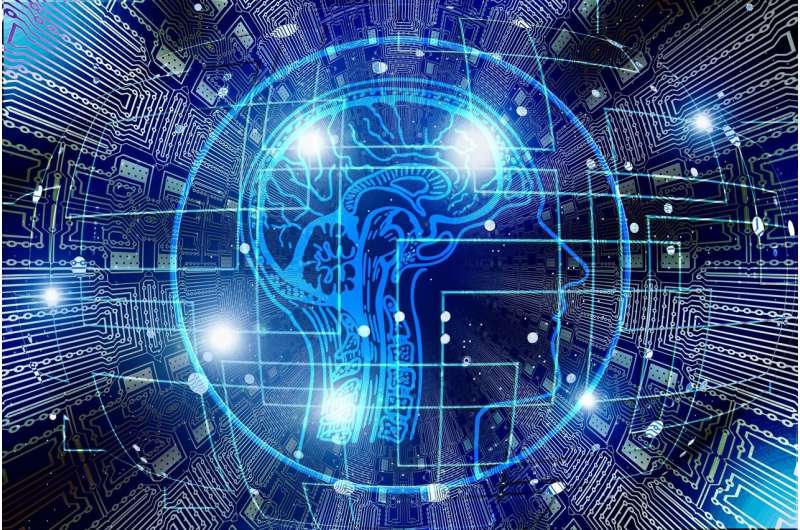
In trying to think of an introduction for this article it occurred to me that had I been inside an MRI, the screen would have showed several brain regions lighting up like Times Square as my mind was attempting to solve the problem.
First, the prefrontal cortex, basal ganglia and thalamus would recognize that the blank page meant that there was a problem that needed to be solved. The thought that the editor might not favor this first-person account in a science article would send the limbic system , the primal part of the brain where emotions are processed, into overdrive. The amygdala, that little almond-shaped nugget at the base of the brain, would look like a Christmas tree as anxiety ticked up.
Finally, as words started filling the screen, the prefrontal cortex behind the forehead would flicker and flash. The hippocampus would access memories of previous similar articles, the information-gathering process and even school-level English classes decades ago, to help the process along. And all this activity would happen at once.
Holistic problem solving
Depending on the problem in front of you, the entire brain could be involved in trying to find a solution, says Professor Kate Cockcroft, Division Leader of cognitive neuroscience at the Neuroscience Research Laboratory (Wits NeuRL) in the School of Human and Community Development.
"You would use many different brain regions to solve a problem, especially a novel or difficult one. The idea of processes being localized in one or two parts of the brain has been replaced with newer evidence that it is the connections among brain areas and their interaction that is important in cognitive processes . Some areas may be more activated with certain problems—a visual problem would activate the visual cortices, for example.
"All this activity takes place as electrochemical signals. The signals form within neurons, pass along the branch-like axons and jump from one neuron to the next across gaps called synapses, with the help of neurotransmitter chemicals. The pattern, size, shape and number of these signals, what they communicate with, and the region of the brain in which they happen, determine what they achieve."
Although problem solving is a metacognitive—"thinking about thinking"—process, that does not make it solely the domain of the highly evolved human prefrontal cortex , adds Dr. Sahba Besharati, Division Leader of social-affective neuroscience at NeuRL.
"This is the most recently evolved part of the human brain, but problem solving does not happen in isolation—it's immersed in a social context that influences how we interpret information. Your background, gender, religion or emotions, among other factors, all influence how you interpret a problem. This means that it would involve other brain areas like the limbic system, one of the oldest brain systems housed deep within the cortex," says Besharati.
"Problem-solving abilities are not a human peculiarity. Some animals are even better than us at solving certain problems, but we all share basic problem-solving skills—if there's danger, leave; if you're hungry, find food."
None of this would be possible without memory either, says Cockcroft. "Without it, we would forget what it is that we are trying to solve and we wouldn't be able to use past experiences to help us solve it."
And memory is, again, linked to emotion. "We use this information to increase the likelihood of positive results when solving new problems," she says.
Improving your skills
It has been proven time and again that just about any brain process can be improved—including problem-solving abilities. "Brain plasticity is a real thing—the brain can reorganize itself with targeted intervention," says Besharati. "Rehabilitation from neurological injury is a dynamic process and an ever-improving science that has allowed us to understand how the brain can change and adapt in response to the environment. Studies have also shown that simple memorisation exercises can assist tremendously in retaining cognitive skills in old age."
Of course, all these processes depend on your brain recognizing that there's a problem to be dealt with in the first place—if you don't realize you're spending money foolishly, you can't improve your finances. "Recognition of a problem can happen at both a conscious and unconscious level. Stroke patients who are not aware of their motor paralysis, for example, deludedly don't believe that they are paralyzed and will sometimes not engage in rehabilitation. But their delusions often spontaneously recover, suggesting recognition at an unconscious level and that, over time, the brain can restore function."
If all else fails, there might be some value to the adage "sleep on it," says Cockcroft. "Sleep is believed to assist memory consolidation—changing memories from a fragile state in which they can easily be damaged to a permanent state. In doing so, they become stored in different brain regions and new neural connections are formed that may assist problem solving. On waking, you may have formed associations between information that you didn't think of previously. This seems to be most effective within three hours of learning new information—perhaps we should institute compulsory naps for students after lectures!"
Explore further
Feedback to editors

Modular communicative leadless ICD found to be safe and exceeds performance expectations
10 hours ago

Creativity and humor shown to promote well-being in older adults via similar mechanisms

Sweet taste receptor affects how glucose is handled metabolically by humans
12 hours ago

Better medical record-keeping needed to fight antibiotic overuse, studies suggest
18 hours ago

Repeat COVID-19 vaccinations elicit antibodies that neutralize variants, other viruses

A long-term ketogenic diet accumulates aged cells in normal tissues, new study shows
May 17, 2024

Gut bacteria enhance cancer immunotherapy in mouse study

Research finds the protein VISTA directly blocks T cells from functioning in immunotherapy

Study opens the door to designing therapies to improve lung development in growth-restricted fetuses

Researchers make strides in microbiome-based cancer therapies via iron deprivation in the tumor microenvironment
Related stories.

Have a vexing problem? Sleep on it.
Oct 17, 2019

Can't solve a riddle? The answer might lie in knowing what doesn't work
Mar 4, 2021

Researchers question the existence of the social brain as a separate system
Oct 15, 2020

Creativity important to lift math education
Feb 4, 2020

A child's brain activity reveals their memory ability
May 25, 2020

Why people with depression can sometimes experience memory problems
Feb 9, 2021
Recommended for you

The neural signature of subjective disgust could apply to both sensory and socio-moral experiences

Researcher discovers drug that may delay onset of Alzheimer's, Parkinson's disease and treat hydrocephalus

Study reveals that the brain modulates visual signals according to internal states

Key protein behind brain asymmetry uncovered by scientists

New technique to freeze brain tissue without harm
Let us know if there is a problem with our content.
Use this form if you have come across a typo, inaccuracy or would like to send an edit request for the content on this page. For general inquiries, please use our contact form . For general feedback, use the public comments section below (please adhere to guidelines ).
Please select the most appropriate category to facilitate processing of your request
Thank you for taking time to provide your feedback to the editors.
Your feedback is important to us. However, we do not guarantee individual replies due to the high volume of messages.
E-mail the story
Your email address is used only to let the recipient know who sent the email. Neither your address nor the recipient's address will be used for any other purpose. The information you enter will appear in your e-mail message and is not retained by Medical Xpress in any form.
Newsletter sign up
Get weekly and/or daily updates delivered to your inbox. You can unsubscribe at any time and we'll never share your details to third parties.
More information Privacy policy
Donate and enjoy an ad-free experience
We keep our content available to everyone. Consider supporting Science X's mission by getting a premium account.
E-mail newsletter
Masks Strongly Recommended but Not Required in Maryland, Starting Immediately
Due to the downward trend in respiratory viruses in Maryland, masking is no longer required but remains strongly recommended in Johns Hopkins Medicine clinical locations in Maryland. Read more .
- Vaccines
- Masking Guidelines
- Visitor Guidelines
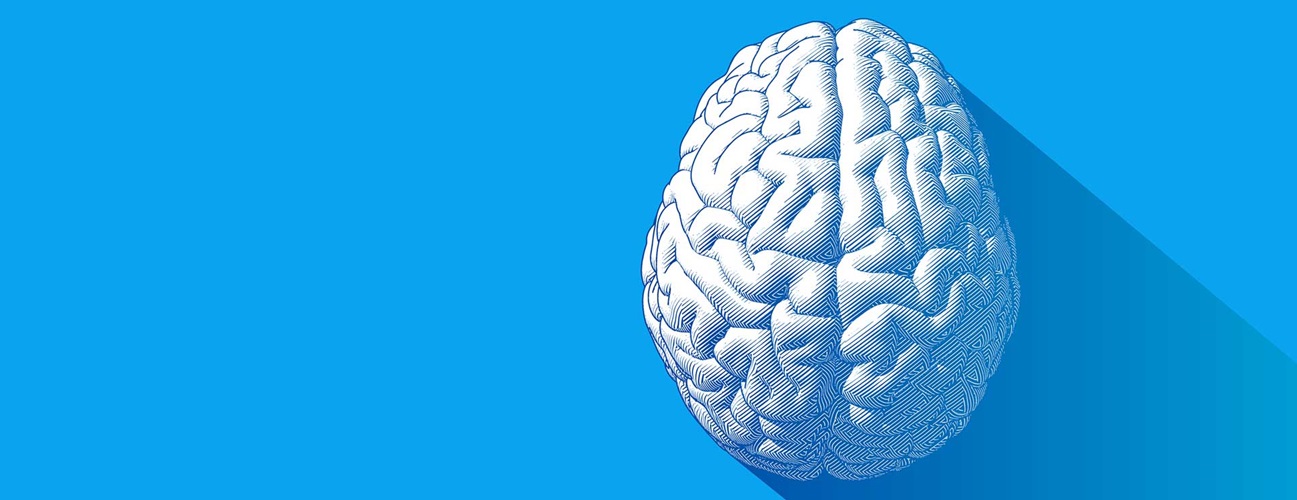
Brain Anatomy and How the Brain Works
What is the brain.
The brain is a complex organ that controls thought, memory, emotion, touch, motor skills, vision, breathing, temperature, hunger and every process that regulates our body. Together, the brain and spinal cord that extends from it make up the central nervous system, or CNS.
What is the brain made of?
Weighing about 3 pounds in the average adult, the brain is about 60% fat. The remaining 40% is a combination of water, protein, carbohydrates and salts. The brain itself is a not a muscle. It contains blood vessels and nerves, including neurons and glial cells.
What is the gray matter and white matter?
Gray and white matter are two different regions of the central nervous system. In the brain, gray matter refers to the darker, outer portion, while white matter describes the lighter, inner section underneath. In the spinal cord, this order is reversed: The white matter is on the outside, and the gray matter sits within.
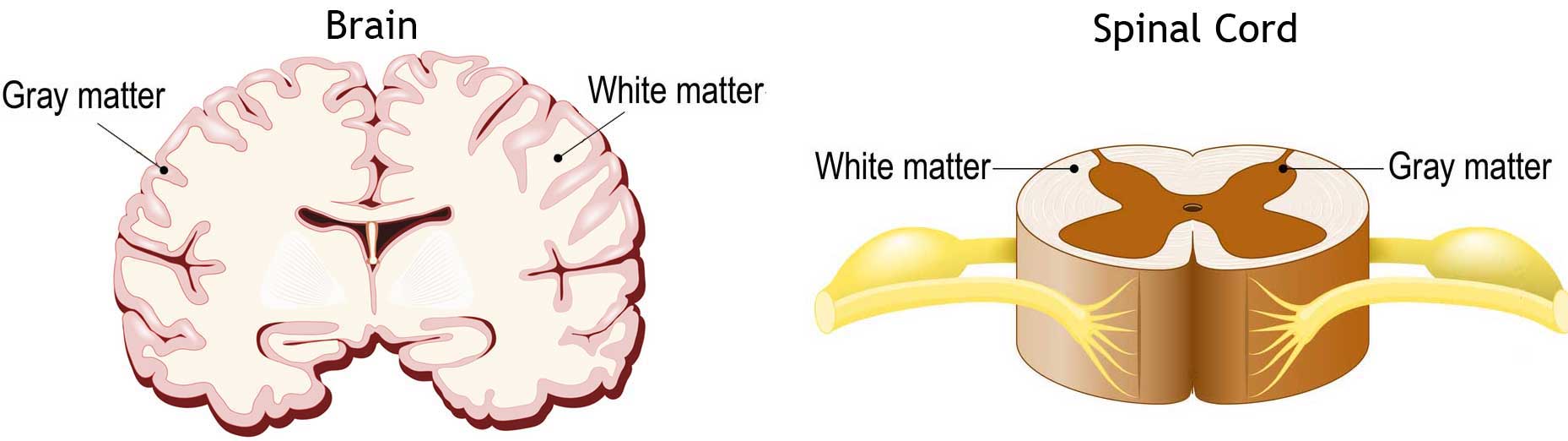
Gray matter is primarily composed of neuron somas (the round central cell bodies), and white matter is mostly made of axons (the long stems that connects neurons together) wrapped in myelin (a protective coating). The different composition of neuron parts is why the two appear as separate shades on certain scans.
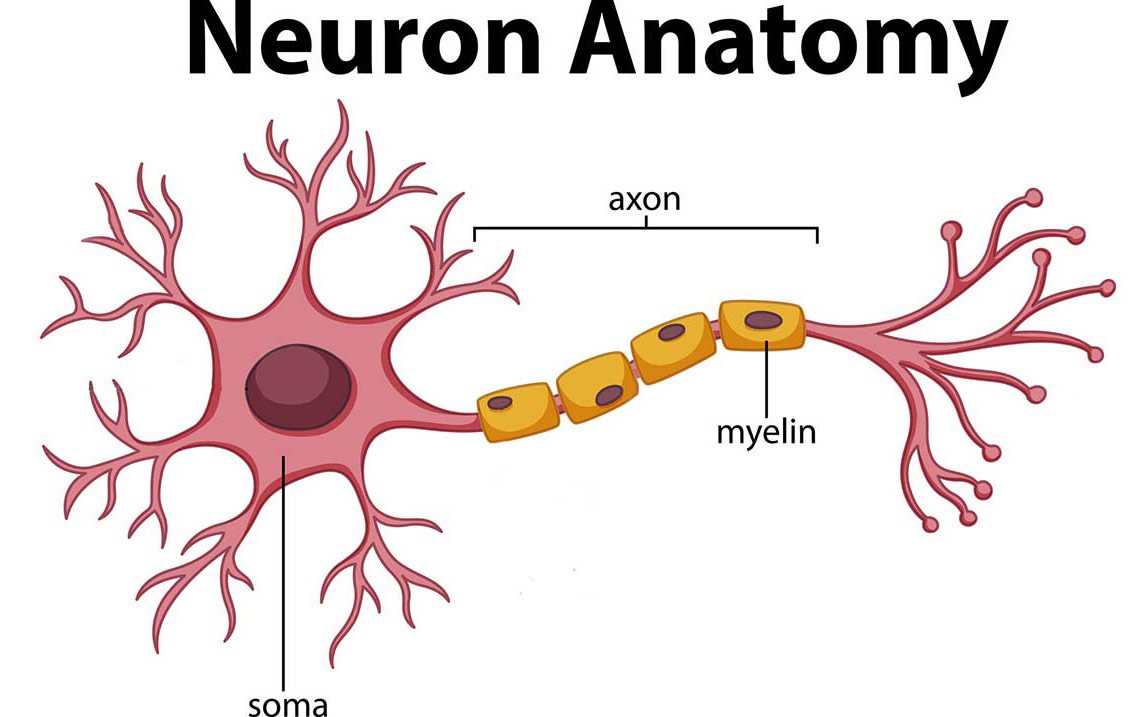
Each region serves a different role. Gray matter is primarily responsible for processing and interpreting information, while white matter transmits that information to other parts of the nervous system.
How does the brain work?
The brain sends and receives chemical and electrical signals throughout the body. Different signals control different processes, and your brain interprets each. Some make you feel tired, for example, while others make you feel pain.
Some messages are kept within the brain, while others are relayed through the spine and across the body’s vast network of nerves to distant extremities. To do this, the central nervous system relies on billions of neurons (nerve cells).
Main Parts of the Brain and Their Functions
At a high level, the brain can be divided into the cerebrum, brainstem and cerebellum.
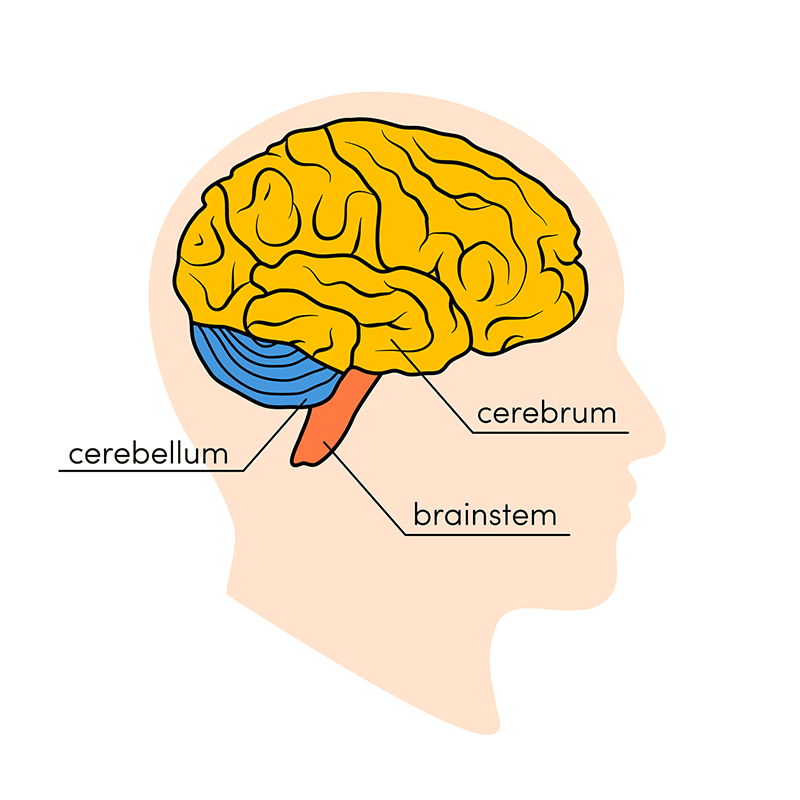
The cerebrum (front of brain) comprises gray matter (the cerebral cortex) and white matter at its center. The largest part of the brain, the cerebrum initiates and coordinates movement and regulates temperature. Other areas of the cerebrum enable speech, judgment, thinking and reasoning, problem-solving, emotions and learning. Other functions relate to vision, hearing, touch and other senses.
Cerebral Cortex
Cortex is Latin for “bark,” and describes the outer gray matter covering of the cerebrum. The cortex has a large surface area due to its folds, and comprises about half of the brain’s weight.
The cerebral cortex is divided into two halves, or hemispheres. It is covered with ridges (gyri) and folds (sulci). The two halves join at a large, deep sulcus (the interhemispheric fissure, AKA the medial longitudinal fissure) that runs from the front of the head to the back. The right hemisphere controls the left side of the body, and the left half controls the right side of the body. The two halves communicate with one another through a large, C-shaped structure of white matter and nerve pathways called the corpus callosum. The corpus callosum is in the center of the cerebrum.
The brainstem (middle of brain) connects the cerebrum with the spinal cord. The brainstem includes the midbrain, the pons and the medulla.
- Midbrain. The midbrain (or mesencephalon) is a very complex structure with a range of different neuron clusters (nuclei and colliculi), neural pathways and other structures. These features facilitate various functions, from hearing and movement to calculating responses and environmental changes. The midbrain also contains the substantia nigra, an area affected by Parkinson’s disease that is rich in dopamine neurons and part of the basal ganglia, which enables movement and coordination.
- Pons. The pons is the origin for four of the 12 cranial nerves, which enable a range of activities such as tear production, chewing, blinking, focusing vision, balance, hearing and facial expression. Named for the Latin word for “bridge,” the pons is the connection between the midbrain and the medulla.
- Medulla. At the bottom of the brainstem, the medulla is where the brain meets the spinal cord. The medulla is essential to survival. Functions of the medulla regulate many bodily activities, including heart rhythm, breathing, blood flow, and oxygen and carbon dioxide levels. The medulla produces reflexive activities such as sneezing, vomiting, coughing and swallowing.
The spinal cord extends from the bottom of the medulla and through a large opening in the bottom of the skull. Supported by the vertebrae, the spinal cord carries messages to and from the brain and the rest of the body.
The cerebellum (“little brain”) is a fist-sized portion of the brain located at the back of the head, below the temporal and occipital lobes and above the brainstem. Like the cerebral cortex, it has two hemispheres. The outer portion contains neurons, and the inner area communicates with the cerebral cortex. Its function is to coordinate voluntary muscle movements and to maintain posture, balance and equilibrium. New studies are exploring the cerebellum’s roles in thought, emotions and social behavior, as well as its possible involvement in addiction, autism and schizophrenia.
Brain Coverings: Meninges
Three layers of protective covering called meninges surround the brain and the spinal cord.
- The outermost layer, the dura mater , is thick and tough. It includes two layers: The periosteal layer of the dura mater lines the inner dome of the skull (cranium) and the meningeal layer is below that. Spaces between the layers allow for the passage of veins and arteries that supply blood flow to the brain.
- The arachnoid mater is a thin, weblike layer of connective tissue that does not contain nerves or blood vessels. Below the arachnoid mater is the cerebrospinal fluid, or CSF. This fluid cushions the entire central nervous system (brain and spinal cord) and continually circulates around these structures to remove impurities.
- The pia mater is a thin membrane that hugs the surface of the brain and follows its contours. The pia mater is rich with veins and arteries.
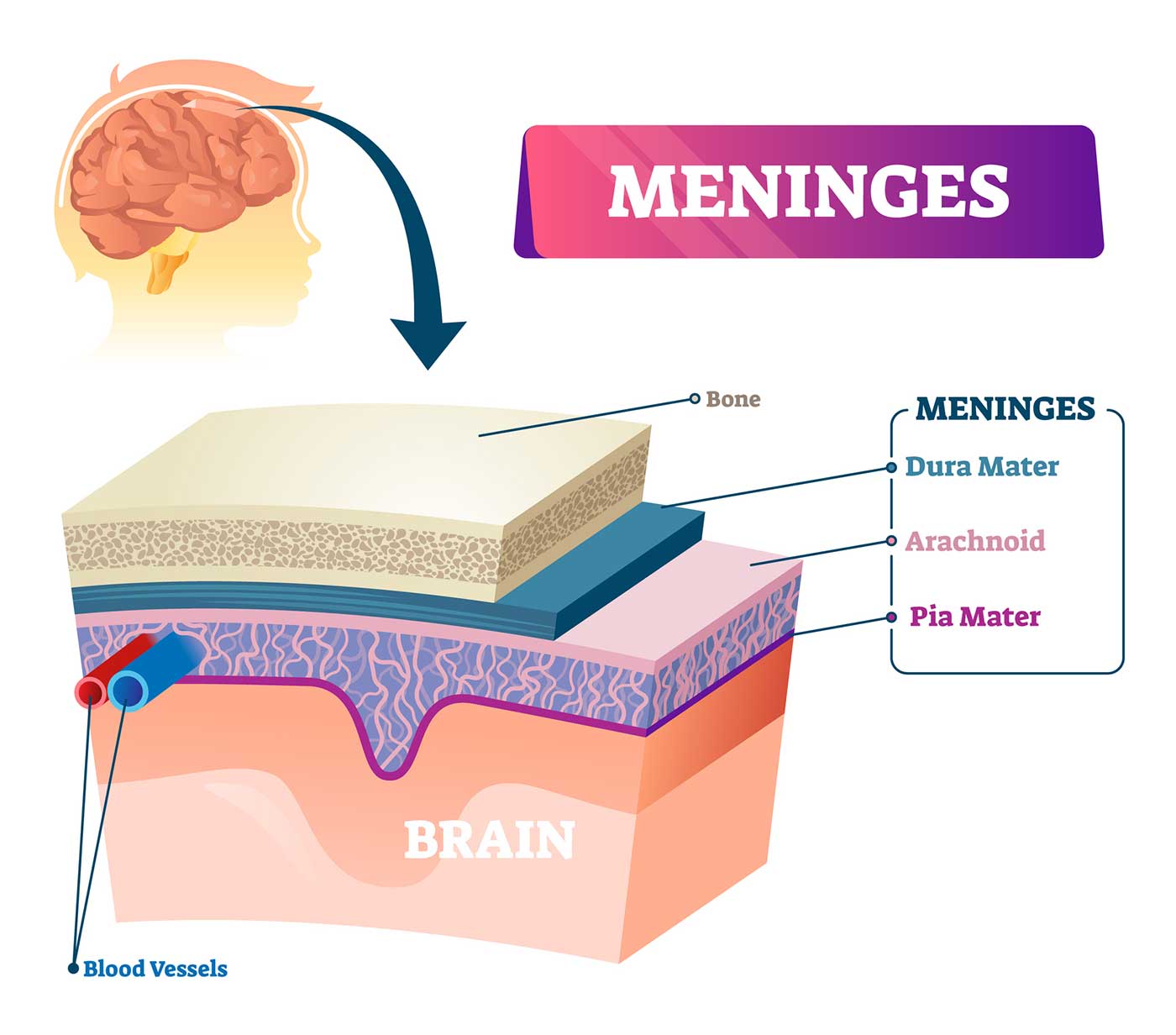
Lobes of the Brain and What They Control
Each brain hemisphere (parts of the cerebrum) has four sections, called lobes: frontal, parietal, temporal and occipital. Each lobe controls specific functions.
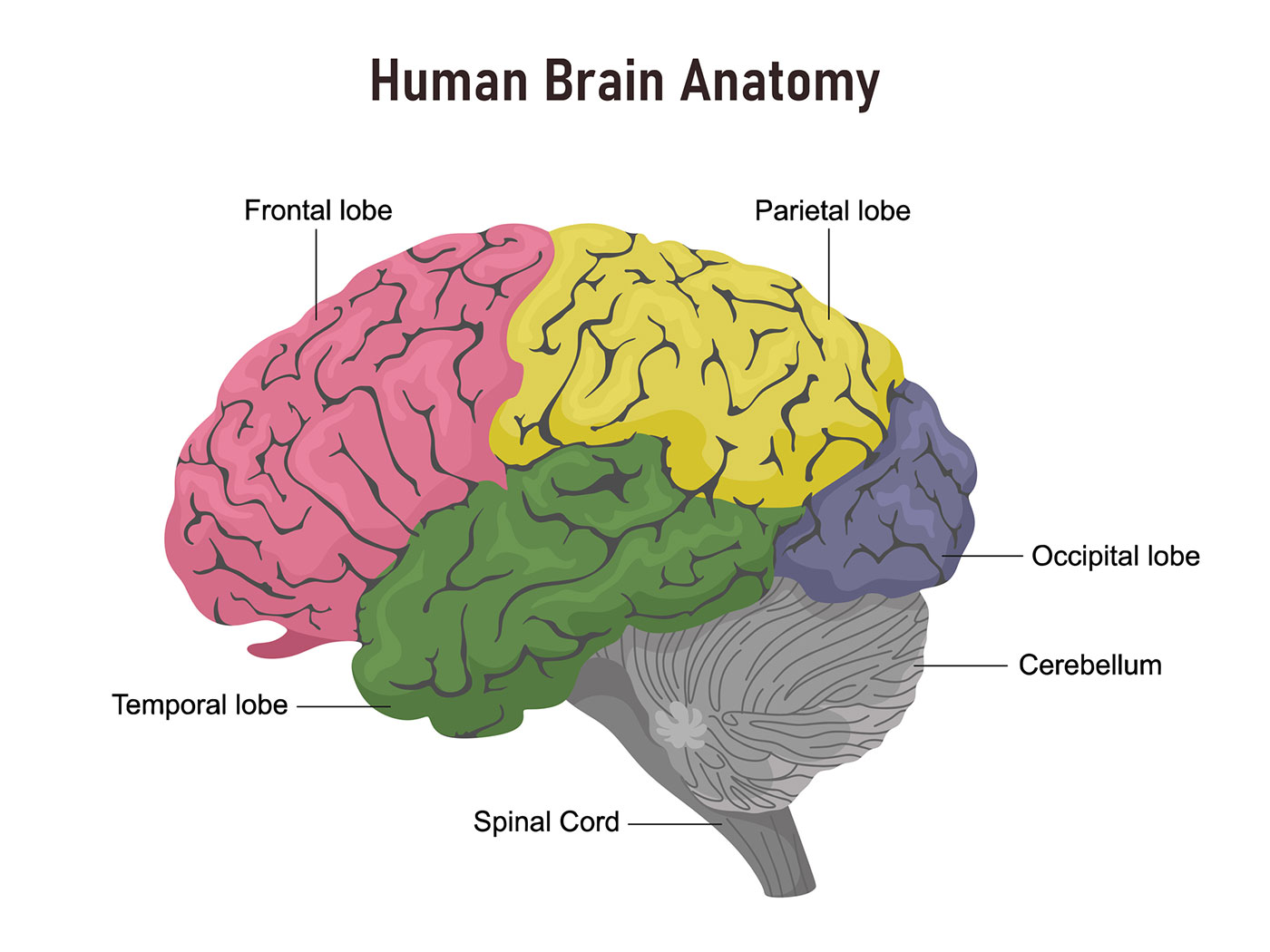
- Frontal lobe. The largest lobe of the brain, located in the front of the head, the frontal lobe is involved in personality characteristics, decision-making and movement. Recognition of smell usually involves parts of the frontal lobe. The frontal lobe contains Broca’s area, which is associated with speech ability.
- Parietal lobe. The middle part of the brain, the parietal lobe helps a person identify objects and understand spatial relationships (where one’s body is compared with objects around the person). The parietal lobe is also involved in interpreting pain and touch in the body. The parietal lobe houses Wernicke’s area, which helps the brain understand spoken language.
- Occipital lobe. The occipital lobe is the back part of the brain that is involved with vision.
- Temporal lobe. The sides of the brain, temporal lobes are involved in short-term memory, speech, musical rhythm and some degree of smell recognition.
Deeper Structures Within the Brain
Pituitary gland.
Sometimes called the “master gland,” the pituitary gland is a pea-sized structure found deep in the brain behind the bridge of the nose. The pituitary gland governs the function of other glands in the body, regulating the flow of hormones from the thyroid, adrenals, ovaries and testicles. It receives chemical signals from the hypothalamus through its stalk and blood supply.
Hypothalamus
The hypothalamus is located above the pituitary gland and sends it chemical messages that control its function. It regulates body temperature, synchronizes sleep patterns, controls hunger and thirst and also plays a role in some aspects of memory and emotion.
Small, almond-shaped structures, an amygdala is located under each half (hemisphere) of the brain. Included in the limbic system, the amygdalae regulate emotion and memory and are associated with the brain’s reward system, stress, and the “fight or flight” response when someone perceives a threat.
Hippocampus
A curved seahorse-shaped organ on the underside of each temporal lobe, the hippocampus is part of a larger structure called the hippocampal formation. It supports memory, learning, navigation and perception of space. It receives information from the cerebral cortex and may play a role in Alzheimer’s disease.
Pineal Gland
The pineal gland is located deep in the brain and attached by a stalk to the top of the third ventricle. The pineal gland responds to light and dark and secretes melatonin, which regulates circadian rhythms and the sleep-wake cycle.
Ventricles and Cerebrospinal Fluid
Deep in the brain are four open areas with passageways between them. They also open into the central spinal canal and the area beneath arachnoid layer of the meninges.
The ventricles manufacture cerebrospinal fluid , or CSF, a watery fluid that circulates in and around the ventricles and the spinal cord, and between the meninges. CSF surrounds and cushions the spinal cord and brain, washes out waste and impurities, and delivers nutrients.
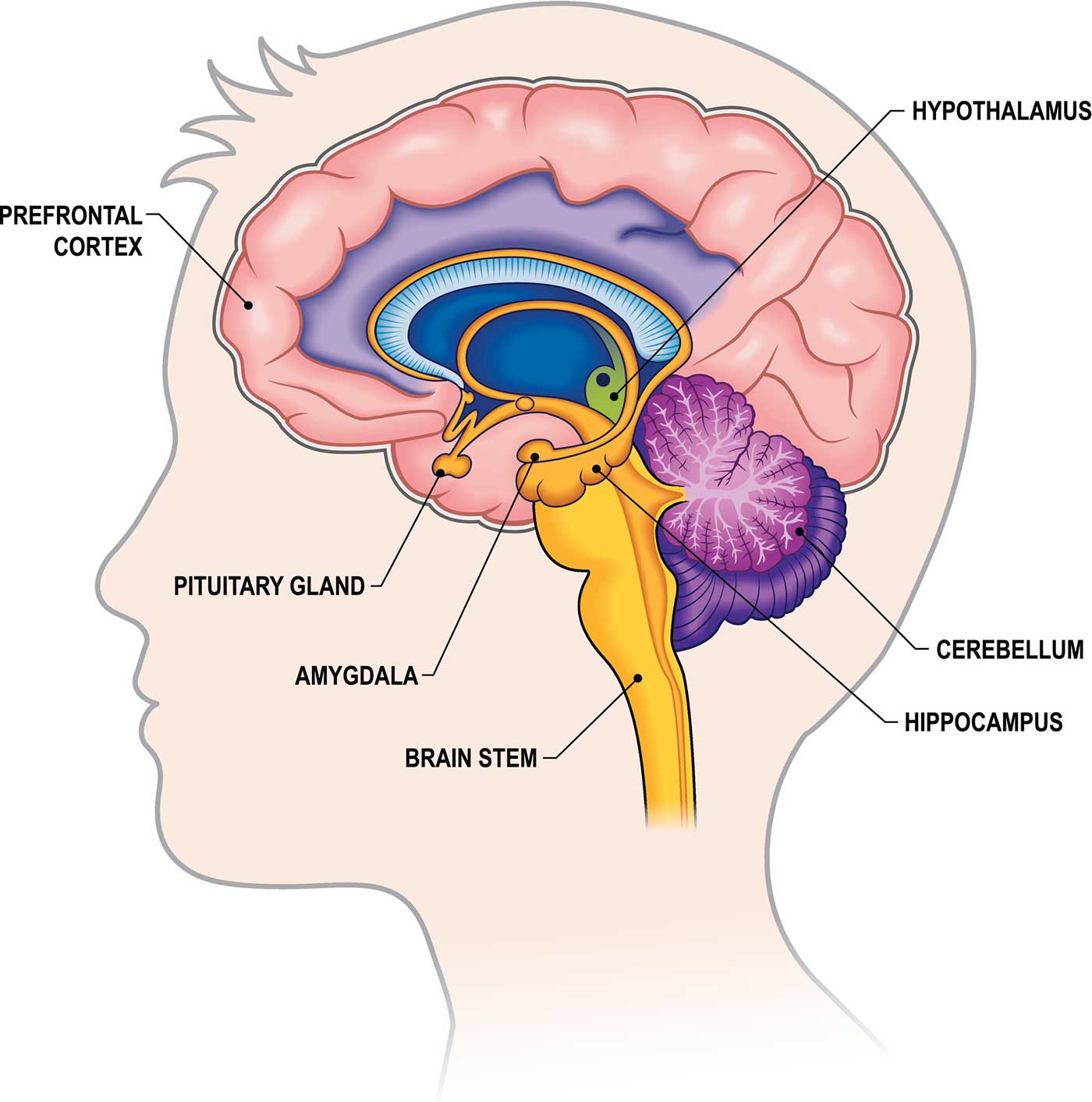
Blood Supply to the Brain
Two sets of blood vessels supply blood and oxygen to the brain: the vertebral arteries and the carotid arteries.
The external carotid arteries extend up the sides of your neck, and are where you can feel your pulse when you touch the area with your fingertips. The internal carotid arteries branch into the skull and circulate blood to the front part of the brain.
The vertebral arteries follow the spinal column into the skull, where they join together at the brainstem and form the basilar artery , which supplies blood to the rear portions of the brain.
The circle of Willis , a loop of blood vessels near the bottom of the brain that connects major arteries, circulates blood from the front of the brain to the back and helps the arterial systems communicate with one another.
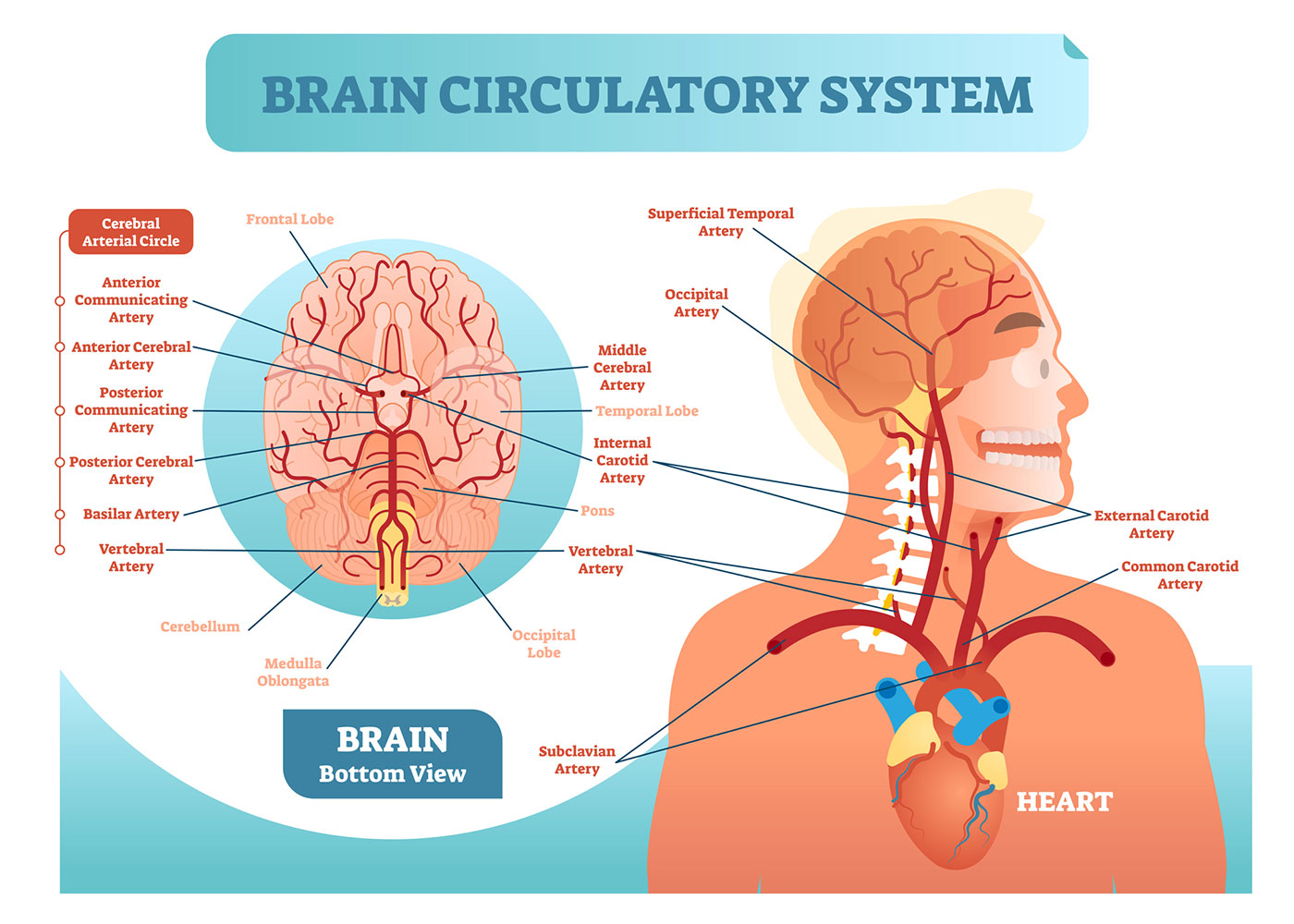
Cranial Nerves
Inside the cranium (the dome of the skull), there are 12 nerves, called cranial nerves:
- Cranial nerve 1: The first is the olfactory nerve, which allows for your sense of smell.
- Cranial nerve 2: The optic nerve governs eyesight.
- Cranial nerve 3: The oculomotor nerve controls pupil response and other motions of the eye, and branches out from the area in the brainstem where the midbrain meets the pons.
- Cranial nerve 4: The trochlear nerve controls muscles in the eye. It emerges from the back of the midbrain part of the brainstem.
- Cranial nerve 5: The trigeminal nerve is the largest and most complex of the cranial nerves, with both sensory and motor function. It originates from the pons and conveys sensation from the scalp, teeth, jaw, sinuses, parts of the mouth and face to the brain, allows the function of chewing muscles, and much more.
- Cranial nerve 6: The abducens nerve innervates some of the muscles in the eye.
- Cranial nerve 7: The facial nerve supports face movement, taste, glandular and other functions.
- Cranial nerve 8: The vestibulocochlear nerve facilitates balance and hearing.
- Cranial nerve 9: The glossopharyngeal nerve allows taste, ear and throat movement, and has many more functions.
- Cranial nerve 10: The vagus nerve allows sensation around the ear and the digestive system and controls motor activity in the heart, throat and digestive system.
- Cranial nerve 11: The accessory nerve innervates specific muscles in the head, neck and shoulder.
- Cranial nerve 12: The hypoglossal nerve supplies motor activity to the tongue.
The first two nerves originate in the cerebrum, and the remaining 10 cranial nerves emerge from the brainstem, which has three parts: the midbrain, the pons and the medulla.
Find a Treatment Center
- Neurology and Neurosurgery
Find Additional Treatment Centers at:
- Howard County Medical Center
- Sibley Memorial Hospital
- Suburban Hospital
Request an Appointment
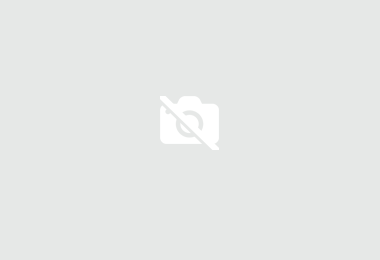
Facioscapulohumeral Muscular Dystrophy in Children
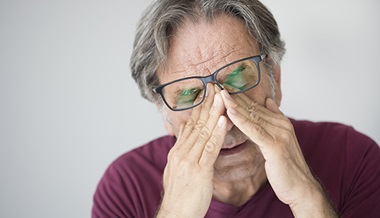
Moyamoya Disease
Motor Stereotypies
Related Topics

- Science Notes Posts
- Contact Science Notes
- Todd Helmenstine Biography
- Anne Helmenstine Biography
- Free Printable Periodic Tables (PDF and PNG)
- Periodic Table Wallpapers
- Interactive Periodic Table
- Periodic Table Posters
- How to Grow Crystals
- Chemistry Projects
- Fire and Flames Projects
- Holiday Science
- Chemistry Problems With Answers
- Physics Problems
- Unit Conversion Example Problems
- Chemistry Worksheets
- Biology Worksheets
- Periodic Table Worksheets
- Physical Science Worksheets
- Science Lab Worksheets
- My Amazon Books
Parts of the Brain and Their Functions

The human brain is the epicenter of our nervous system and plays a pivotal role in virtually every aspect of our lives. It’s a complex, highly organized organ responsible for thoughts, feelings, actions, and interactions with the world around us. Here is a look at the intricate anatomy of the brain, its functions, and the consequences of damage to different areas.
Introduction to the Brain and Its Functions
The brain is an organ of soft nervous tissue that is protected within the skull of vertebrates. It functions as the coordinating center of sensation and intellectual and nervous activity. The brain consists of billions of neurons (nerve cells) that communicate through intricate networks. The primary functions of the brain include processing sensory information, regulating bodily functions, forming thoughts and emotions, and storing memories.
Main Parts of the Brain – Anatomy
The three main parts of the brain are the cerebrum, cerebellum, and brainstem.
1. Cerebrum
- Location: The cerebellum occupies the upper part of the cranial cavity and is the largest part of the human brain.
- Functions: It’s responsible for higher brain functions, including thought, action, emotion, and interpretation of sensory data.
- Effects of Damage: Depending on the area affected, damage leads to memory loss, impaired cognitive skills, changes in personality, and loss of motor control.
2. Cerebellum
- Location: The cerebellum is at the back of the brain, below the cerebrum.
- Functions: It coordinates voluntary movements such as posture, balance, coordination, and speech.
- Effects of Damage: Damage causes problems with balance, movement, and muscle coordination (ataxia).
3. Brainstem
- Location: The brainstem is lower extension of the brain, connecting to the spinal cord. It includes the midbrain, pons, and medulla oblongata.
- Functions: This part of the brain controls many basic life-sustaining functions, including heart rate, breathing, sleeping, and eating.
- Effects of Damage: Damage results in life-threatening conditions like breathing difficulties, heart problems, and loss of consciousness.
Lobes of the Brain
The four lobes of the brain are regions of the cerebrum:
- Location: This is the anterior or front part of the brain.
- Functions: Decision making, problem solving, control of purposeful behaviors, consciousness, and emotions.
- Location: Sits behind the frontal lobe.
- Functions: Processes sensory information it receives from the outside world, mainly relating to spatial sense and navigation (proprioception).
- Location: Below the lateral fissure, on both cerebral hemispheres.
- Functions: Mainly revolves around auditory perception and is also important for the processing of both speech and vision (reading).
- Location: At the back of the brain.
- Functions: Main center for visual processing.
Left vs. Right Brain Hemispheres
The cerebrum has two halves, called hemispheres. Each half controls functions on the opposite side of the body. So, the left hemisphere controls muscles on the right side of the body, and vice versa. But, the functions of the two hemispheres are not entirely identical:
- Left Hemisphere: It’s dominant in language and speech and plays roles in logical thinking, analysis, and accuracy. .
- Right Hemisphere: This hemisphere is more visual and intuitive and functions in creative and imaginative tasks.
The corpus callosum is a band of nerves that connect the two hemispheres and allow communication between them.
Detailed List of Parts of the Brain
While knowing the three key parts of the brain is a good start, the anatomy is quite a bit more complex. In addition to nervous tissues, the brain also contains key glands:
- Cerebrum: The cerebrum is the largest part of the brain. Divided into lobes, it coordinates thought, movement, memory, senses, speech, and temperature.
- Corpus Callosum : A broad band of nerve fibers joining the two hemispheres of the brain, facilitating interhemispheric communication.
- Cerebellum : Coordinates movement and balance and aids in eye movement.
- Pons : Controls voluntary actions, including swallowing, bladder function, facial expression, posture, and sleep.
- Medulla oblongata : Regulates involuntary actions, including breathing, heart rhythm, as well as oxygen and carbon dioxide levels.
- Limbic System : Includes the amygdala, hippocampus, and parts of the thalamus and hypothalamus.
- Amygdala: Plays a key role in emotional responses, hormonal secretions, and memory formation.
- Hippocampus: Plays a vital role in memory formation and spatial navigation.
- Thalamus : Acts as the brain’s relay station, channeling sensory and motor signals to the cerebral cortex, and regulating consciousness, sleep, and alertness.
- Basal Ganglia : A group of structures involved in processing information related to movement, emotions, and reward. Key structures include the striatum, globus pallidus, substantia nigra, and subthalamic nucleus.
- Ventral Tegmental Area (VTA) : Plays a role in the reward circuit of the brain, releasing dopamine in response to stimuli indicating a reward.
- Optic tectum : Also known as the superior colliculus, it directs eye movements.
- Substantia Nigra : Involved in motor control and contains a large concentration of dopamine-producing neurons.
- Cingulate Gyrus : Plays a role in processing emotions and behavior regulation. It also helps regulate autonomic motor function.
- Olfactory Bulb : Involved in the sense of smell and the integration of olfactory information.
- Mammillary Bodies : Plays a role in recollective memory.
- Function: Regulates emotions, memory, and arousal.
Glands in the Brain
The hypothalamus, pineal gland, and pituitary gland are the three endocrine glands within the brain:
- Hypothalamus : The hypothalamus links the nervous and endocrine systems. It contains many small nuclei. In addition to participating in eating and drinking, sleeping and waking, it regulates the endocrine system via the pituitary gland. It maintains the body’s homeostasis, regulating hunger, thirst, response to pain, levels of pleasure, sexual satisfaction, anger, and aggressive behavior.
- Pituitary Gland : Known as the “master gland,” it controls various other hormone glands in the body, such as the thyroid and adrenals, as well as regulating growth, metabolism, and reproductive processes.
- Pineal Gland : The pineal gland produces and regulates some hormones, including melatonin, which is crucial in regulating sleep patterns and circadian rhythms.
Gray Matter vs. White Matter
The brain and spinal cord consist of gray matter (substantia grisea) and white matter (substantia alba).
- White Matter: Consists mainly of axons and myelin sheaths that send signals between different brain regions and between the brain and spinal cord.
- Gray Matter: Consists of neuronal cell bodies, dendrites, and axon terminals. Gray matter processes information and directs stimuli for muscle control, sensory perception, decision making, and self-control.
Frequently Asked Questions (FAQs) About the Human Brain
- The human brain contains approximately 86 billion neurons. Additionally, it has a similar or slightly higher number of non-neuronal cells (glial cells), making the total number of cells in the brain close to 170 billion.
- There are about 86 billion neurons in the human brain. These neurons are connected by trillions of synapses, forming a complex networks.
- The average adult human brain weighs about 1.3 to 1.4 kilograms (about 3 pounds). This weight represents about 2% of the total body weight.
- The brain is about 73% water.
- The myth that humans only use 10% of their brain is false. Virtually every part gets use, and most of the brain is active all the time, even during sleep.
- The average size of the adult human brain is about 15 centimeters (6 inches) in length, 14 centimeters (5.5 inches) in width, and 9 centimeters (3.5 inches) in height.
- Brain signal speeds vary depending on the type of neuron and the nature of the signal. They travel anywhere from 1 meter per second to over 100 meters per second in the fastest neurons.
- With age, the brain’s volume and/or weight decrease, synaptic connections reduce, and there can be a decline in cognitive functions. However, the brain to continues adapting and forming new connections throughout life.
- The brain has a limited ability to repair itself. Neuroplasticity aids recovery by allowing other parts of the brain to take over functions of the damaged areas.
- The brain consumes about 20% of the body’s total energy , despite only making up about 2% of the body’s total weight . It requires a constant supply of glucose and oxygen.
- Sleep is crucial for brain health. It aids in memory consolidation, learning, brain detoxification, and the regulation of mood and cognitive functions.
- Douglas Fields, R. (2008). “White Matter Matters”. Scientific American . 298 (3): 54–61. doi: 10.1038/scientificamerican0308-54
- Kandel, Eric R.; Schwartz, James Harris; Jessell, Thomas M. (2000). Principles of Neural Science (4th ed.). New York: McGraw-Hill. ISBN 978-0-8385-7701-1.
- Kolb, B.; Whishaw, I.Q. (2003). Fundamentals of Human Neuropsychology (5th ed.). New York: Worth Publishing. ISBN 978-0-7167-5300-1.
- Rajmohan, V.; Mohandas, E. (2007). “The limbic system”. Indian Journal of Psychiatry . 49 (2): 132–139. doi: 10.4103/0019-5545.33264
- Shepherd, G.M. (1994). Neurobiology . Oxford University Press. ISBN 978-0-19-508843-4.
Related Posts
Coronavirus (COVID-19): Latest Updates | Visitation Policies Visitation Policies Visitation Policies Visitation Policies Visitation Policies | COVID-19 Testing | Vaccine Information Vaccine Information Vaccine Information
Health Encyclopedia
Anatomy of the brain, what is the central nervous system (cns).
The CNS consists of the brain and spinal cord. The brain is an important organ that controls thought, memory, emotion, touch, motor skills, vision, breathing, temperature, hunger, and every process that regulates our body. The brain determines your personality and how you interact with the environment, including other people. This defines who you are.
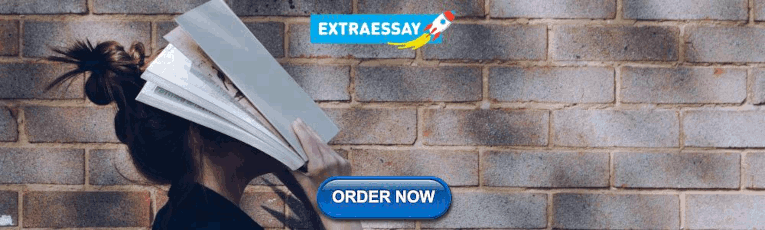
What are the different parts of the brain?
The brain can be divided into the cerebrum, brainstem, and cerebellum:
Cerebrum. This is the front of the brain. It is made up of the right and left hemispheres, which are joined by the corpus callosum. The cerebrum controls: initiation of movement, coordination of movement, temperature, touch, vision, hearing, judgment, reasoning, problem solving, emotions, and learning. The cerebrum is responsible for communication (speaking and writing), memory, abstract thought, and appreciation for music and art.
Brainstem. This is the middle of the brain. It includes the midbrain, the pons, and the medulla. The brainstem controls movement of the eyes, face, and mouth. It also relays sensory messages (such as hot, pain, and loud) and controls respirations, consciousness, cardiac function, involuntary muscle movements, sneezing, coughing, vomiting, and swallowing.
Cerebellum. This is the back of the brain. It coordinates voluntary muscle movements and helps to maintain posture, balance, and equilibrium.
More specifically, other parts of the brain include the following:
Pons. A deep part of the brain, located in the brainstem, the pons contains many of the control areas for eye and face movements.
Medulla. The lowest part of the brainstem, the medulla is the most vital part of the entire brain and contains important control centers for the heart and lungs.
Spinal cord. A large bundle of nerve fibers located in the back that extends from the base of the brain to the lower back, the spinal cord carries messages to and from the brain and the rest of the body.
Frontal lobe. The largest section of the brain located in the front of the head, the frontal lobe is involved in personality characteristics and movement. Recognition of smell often involves parts of the frontal lobe.
Parietal lobe. The middle part of the brain, the parietal lobe helps a person to identify objects and understand spatial relationships (where one's body is compared to objects around the person). The parietal lobe is also involved in interpreting pain and touch in the body.
Occipital lobe. This is the back part of the brain that is involved with vision.
Temporal lobe. The sides of the brain, these temporal lobes are involved in short-term memory, speech, musical rhythm, and some degree of smell recognition. The temporal lobes are also important in understanding sound and voice.
Medical Reviewers:
- Anne Fetterman RN BSN
- Joseph Campellone MD
- Raymond Kent Turley BSN MSN RN
- Ask a Medical Librarian Make an Appointment Physicians & Services Physicians who treat Neurological conditions
Parts of the Brain: Anatomy, Structure & Functions
Olivia Guy-Evans, MSc
Associate Editor for Simply Psychology
BSc (Hons) Psychology, MSc Psychology of Education
Olivia Guy-Evans is a writer and associate editor for Simply Psychology. She has previously worked in healthcare and educational sectors.
Learn about our Editorial Process
Saul Mcleod, PhD
Editor-in-Chief for Simply Psychology
BSc (Hons) Psychology, MRes, PhD, University of Manchester
Saul Mcleod, PhD., is a qualified psychology teacher with over 18 years of experience in further and higher education. He has been published in peer-reviewed journals, including the Journal of Clinical Psychology.
On This Page:
The brain controls all functions of the body, interprets information from the outside world, and defines who we are as individuals and how we experience the world.
The brain receives information through our senses: sight, touch, taste, smell, and hearing. This information is processed in the brain, allowing us to give meaning to the input it receives.
The brain is part of the central nervous system ( CNS ) along with the spinal cord. There is also a peripheral nervous system (PNS) comprised of 31 pairs of spinal nerves that branch from the spinal cord and cranial nerves that branch from the brain.
Brain Parts
The brain is composed of the cerebrum, cerebellum, and brainstem (Fig. 1).
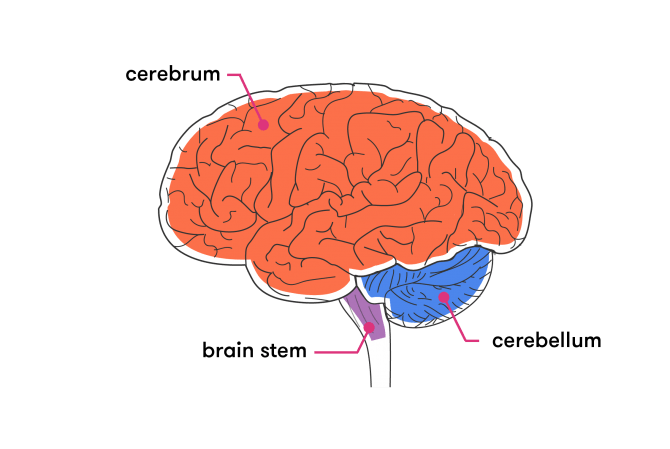
Figure 1. The brain has three main parts: the cerebrum, cerebellum, and brainstem.
The cerebrum is the largest and most recognizable part of the brain. It consists of grey matter (the cerebral cortex ) and white matter at the center. The cerebrum is divided into two hemispheres, the left and right, and contains the lobes of the brain (frontal, temporal, parietal, and occipital lobes).
The cerebrum produces higher functioning roles such as thinking, learning, memory, language, emotion, movement, and perception.
The Cerebellum
The cerebellum is located under the cerebrum and monitors and regulates motor behaviors, especially automatic movements.
This structure is also important for regulating posture and balance and has recently been suggested for being involved in learning and attention.
Although the cerebellum only accounts for roughly 10% of the brain’s total weight, this area is thought to contain more neurons (nerve cells) than the rest of the brain combined.
The brainstem is located at the base of the brain. This area connects the cerebrum and the cerebellum to the spinal cord, acting as a relay station for these areas.
The brainstem regulates automatic functions such as sleep cycles, breathing, body temperature, digestion, coughing, and sneezing.
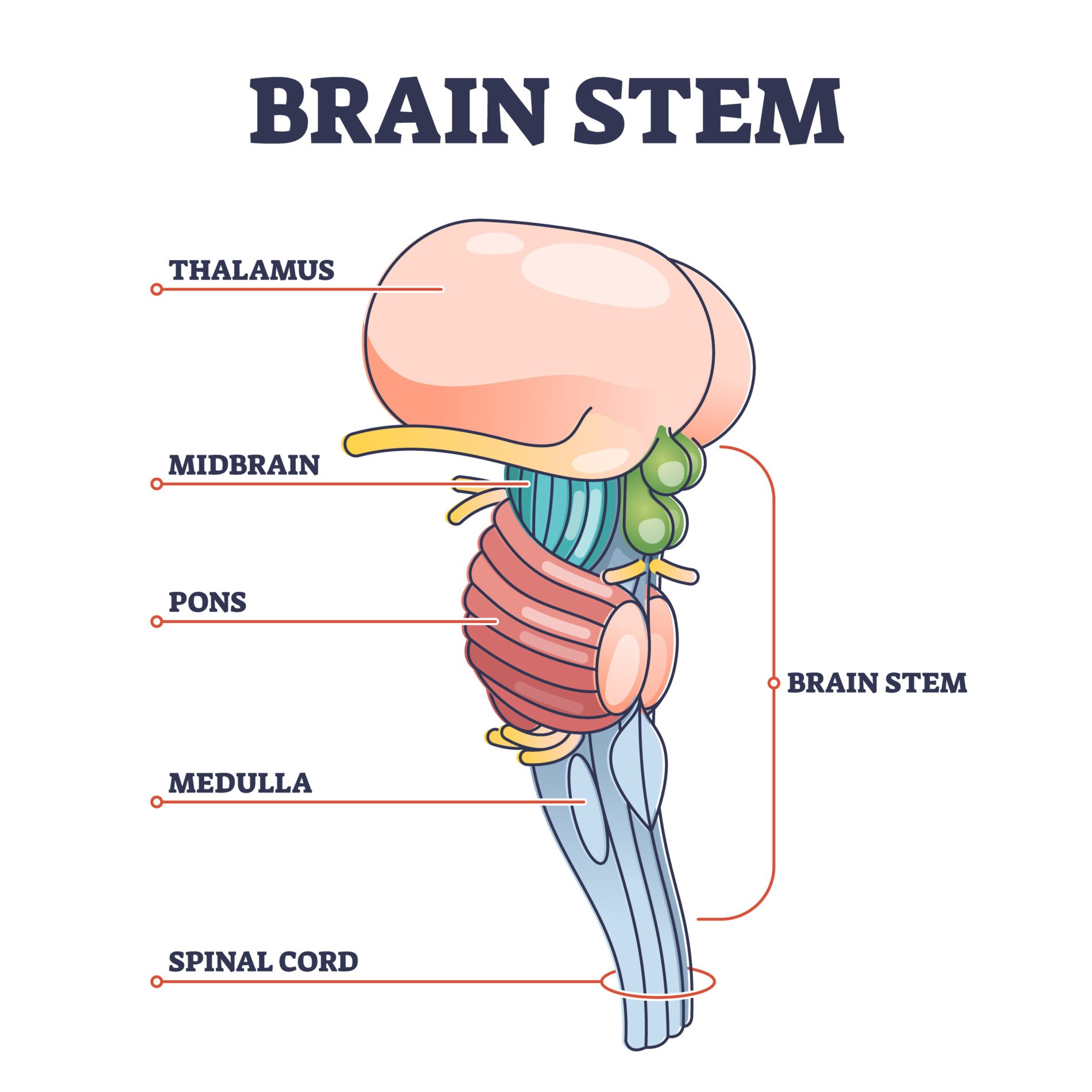
Right Brain vs. Left Brain
The cerebrum is divided into two halves: the right and left hemispheres (Fig. 2). The left hemisphere controls the right half of the body, and the right hemisphere controls the left half.
The two hemispheres are connected by a thick band of neural fibers known as the corpus callosum, consisting of about 200 million axons.
The corpus callosum allows the two hemispheres to communicate and allows information being processed on one side of the brain to be shared with the other.
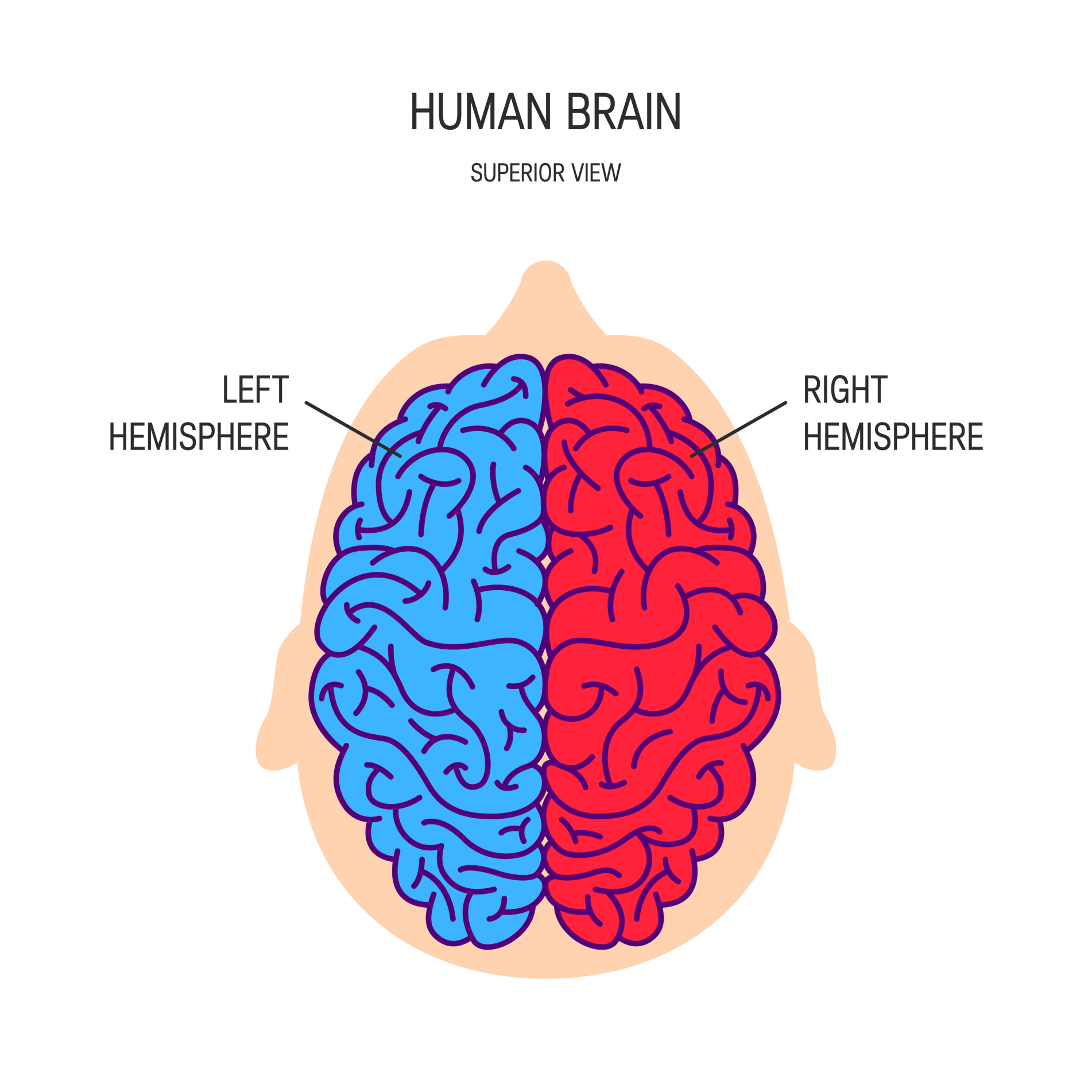
Figure 2. The cerebrum is divided into left and right hemispheres. The nerve fibers corpus callosum connects the two sides.
Hemispheric lateralization is the idea that each hemisphere is responsible for different functions. Each of these functions is localized to either the right or left side.
The left hemisphere is associated with language functions, such as formulating grammar and vocabulary and containing different language centers (Broca’s and Wernicke’s area).
The right hemisphere is associated with more visuospatial functions such as visualization, depth perception, and spatial navigation. These left and right functions are the case in most people, especially those who are right-handed.
Lobes of the Brain
Each cerebral hemisphere can be subdivided into four lobes, each associated with different functions.
The four lobes of the brain are the frontal, parietal, temporal, and occipital lobes (Figure 3).
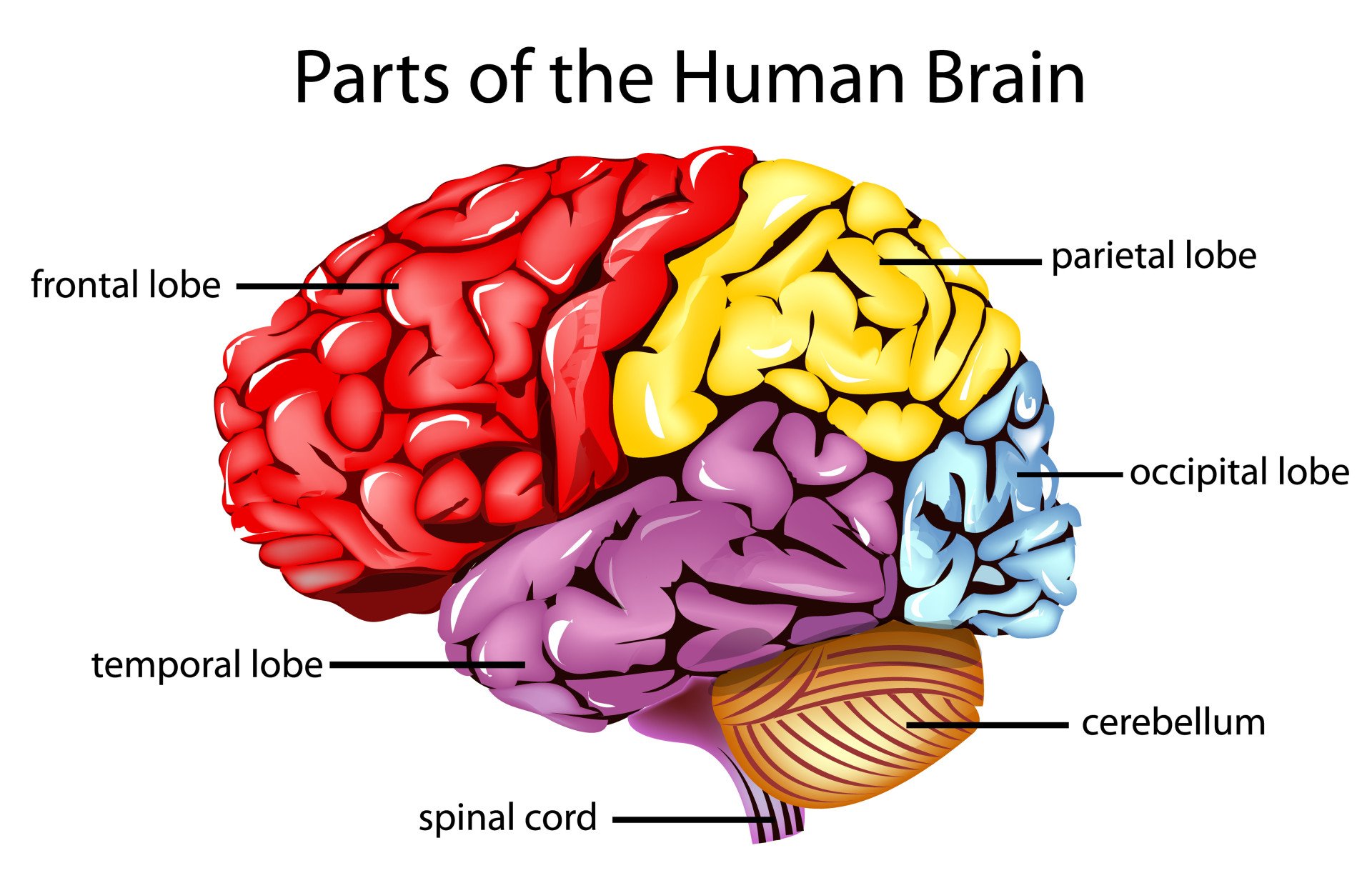
Figure 3. The cerebrum is divided into four lobes: frontal, parietal, occipital, and temporal.
Frontal lobes
The frontal lobes are located at the front of the brain, behind the forehead (Figure 4).
Their main functions are associated with higher cognitive functions, including problem-solving, decision-making, attention, intelligence, and voluntary behaviors.
The frontal lobes contain the motor cortex responsible for planning and coordinating movements.
It also contains the prefrontal cortex, which is responsible for initiating higher-lever cognitive functioning, and Broca’s Area, which is essential for language production.
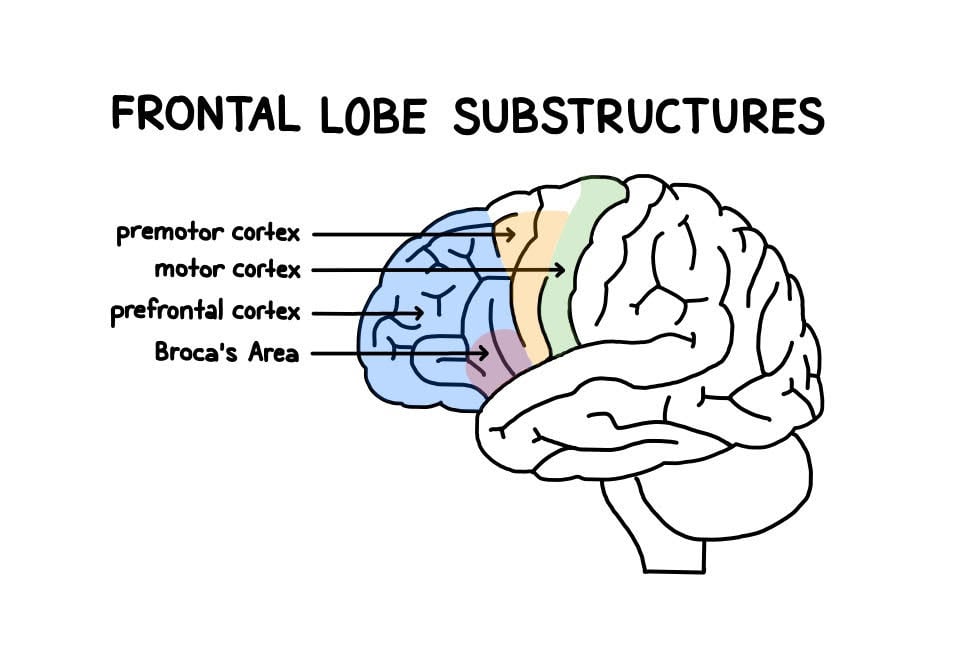
Figure 4. Frontal lobe structure.
Temporal lobes
The temporal lobes are located on both sides of the brain, near the temples of the head, hence the name temporal lobes (Figure 5).
The main functions of these lobes include understanding, language, memory acquisition, face recognition, object recognition, perception, and auditory information processing.
There is a temporal lobe in both the left and right hemispheres. The left temporal lobe, which is usually the most dominant in people, is associated with language, learning, memorizing, forming words, and remembering verbal information.
The left lobe also contains a vital language center known as Wernicke’s area, which is essential for language development. The right temporal lobe is usually associated with learning and memorizing non-verbal information and determining facial expressions.
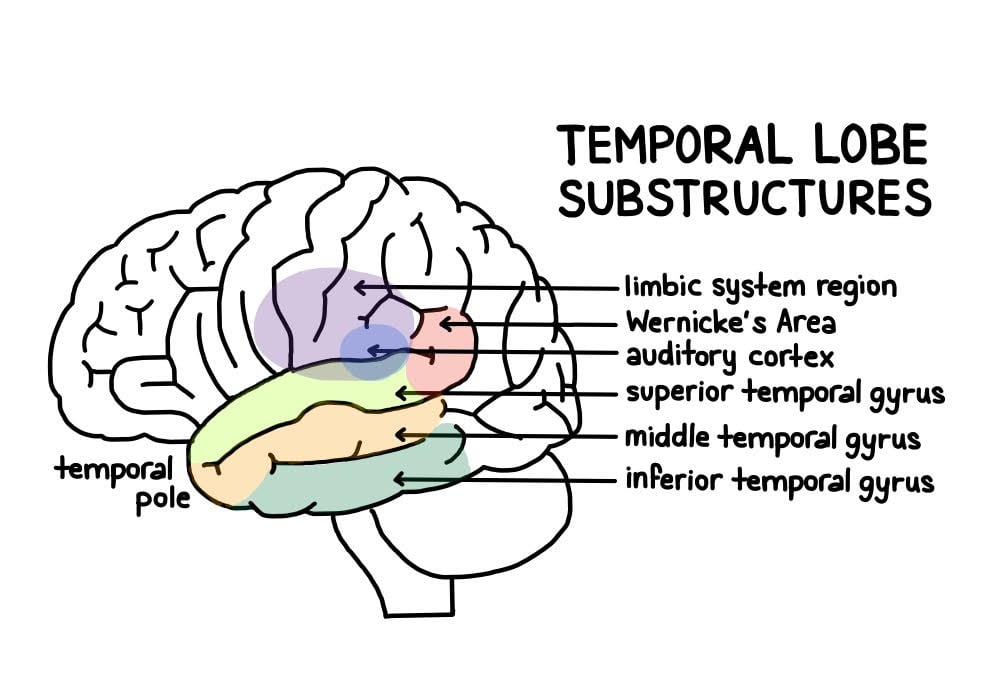
Figure 5. Temporal lobe structure.
Parietal lobes
The parietal lobe is located at the top of the brain, between the frontal and occipital lobes, and above the temporal lobes (Figure 6).
The parietal lobe is essential for integrating information from the body’s senses to allow us to build a coherent picture of the world around us.
These lobes allow us to perceive our bodies through somatosensory information (e.g., through touch, pressure, and temperature). It can also help with visuospatial processing, reading, and number representations (mathematics).
The parietal lobes also contain the somatosensory cortex, which receives and processes sensory information, integrating this into a representational map of the body.
This means it can pinpoint the exact area of the body where a sensation is felt, as well as perceive the weight of objects, shape, and texture.
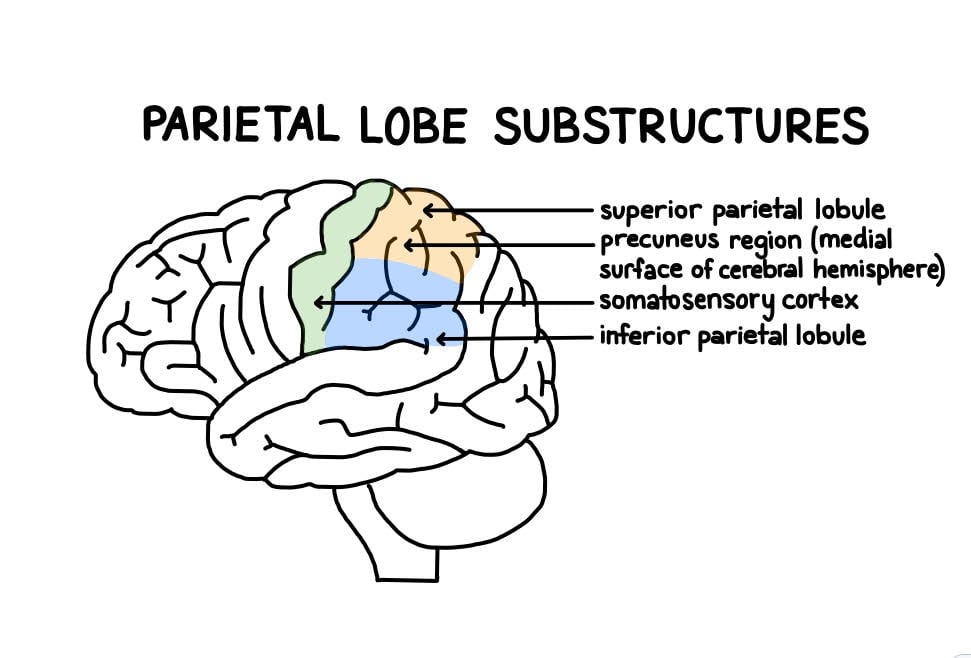
Figure 6. Parietal lobe structure.
Occipital lobes
The occipital lobes are located at the back of the brain behind the temporal and parietal lobes and below the occipital bone of the skull (Figure 7).
The occipital lobes receive sensory information from the eyes’ retinas, which is then encoded into different visual data. Some of the functions of the occipital lobes include being able to assess the size, depth, and distance, determine color information, object and facial recognition, and mapping the visual world.
The occipital lobes also contain the primary visual cortex, which receives sensory information from the retinas, transmitting this information relating to location, spatial data, motion, and the colors of objects in the field of vision.
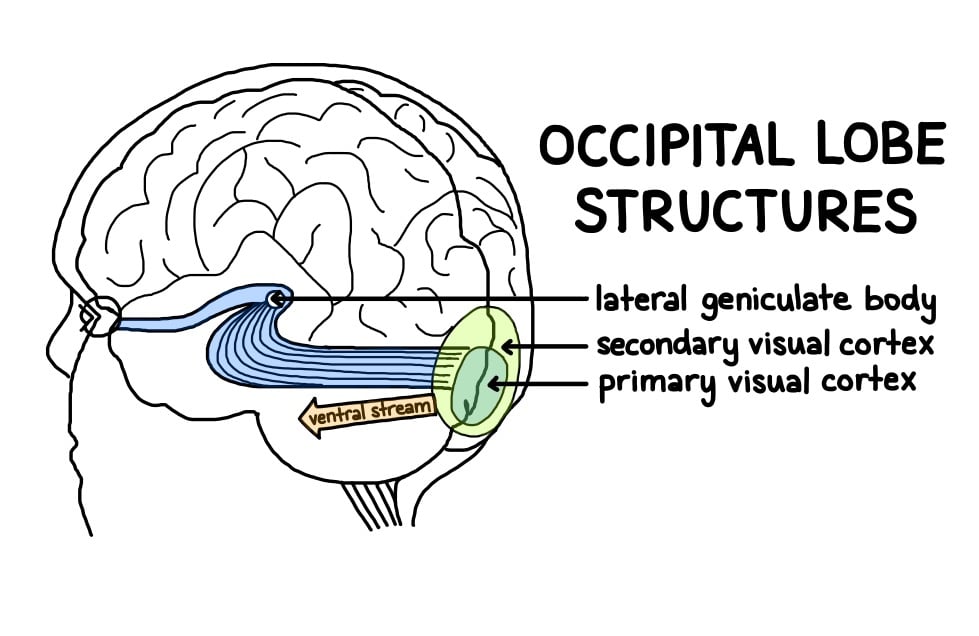
Figure 7. Occipital lobe structure.
Cerebral Cortex
The surface of the cerebrum is called the cerebral cortex and has a wrinkled appearance, consisting of bulges, also known as gyri, and deep furrows, known as sulci (Figure 8).
A gyrus (plural: gyri) is the name given to the bumps and ridges on the cerebral cortex (the outermost layer of the brain). A sulcus (plural: sulci) is another name for a groove in the cerebral cortex.
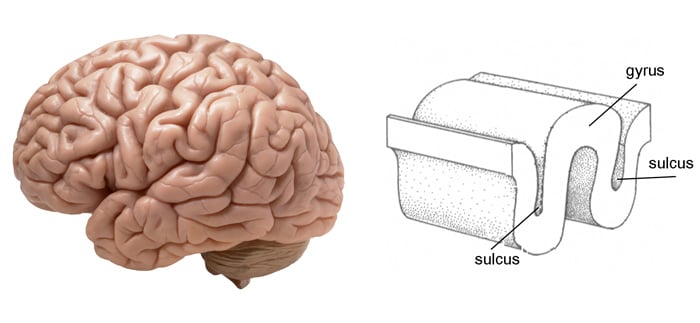
Figure 8. The cortex contains neurons (grey matter) interconnected to other brain areas by axons (white matter). The cortex has a folded appearance. A fold is called a gyrus, and the valley between is a sulcus.
The cerebral cortex is primarily constructed of grey matter (neural tissue made up of neurons), with between 14 and 16 billion neurons found here.
The many folds and wrinkles of the cerebral cortex allow a wider surface area for an increased number of neurons to live there, permitting large amounts of information to be processed.
Deep Structures
The amygdala is a structure deep in the brain that is involved in the processing of emotions and fear learning. The amygdala is a part of the limbic system, a neural network that mediates emotion and memory (Figure 9).
This structure also ties emotional meaning to memories, processes rewards, and helps us make decisions. This structure has also been linked with the fight-or-flight response.
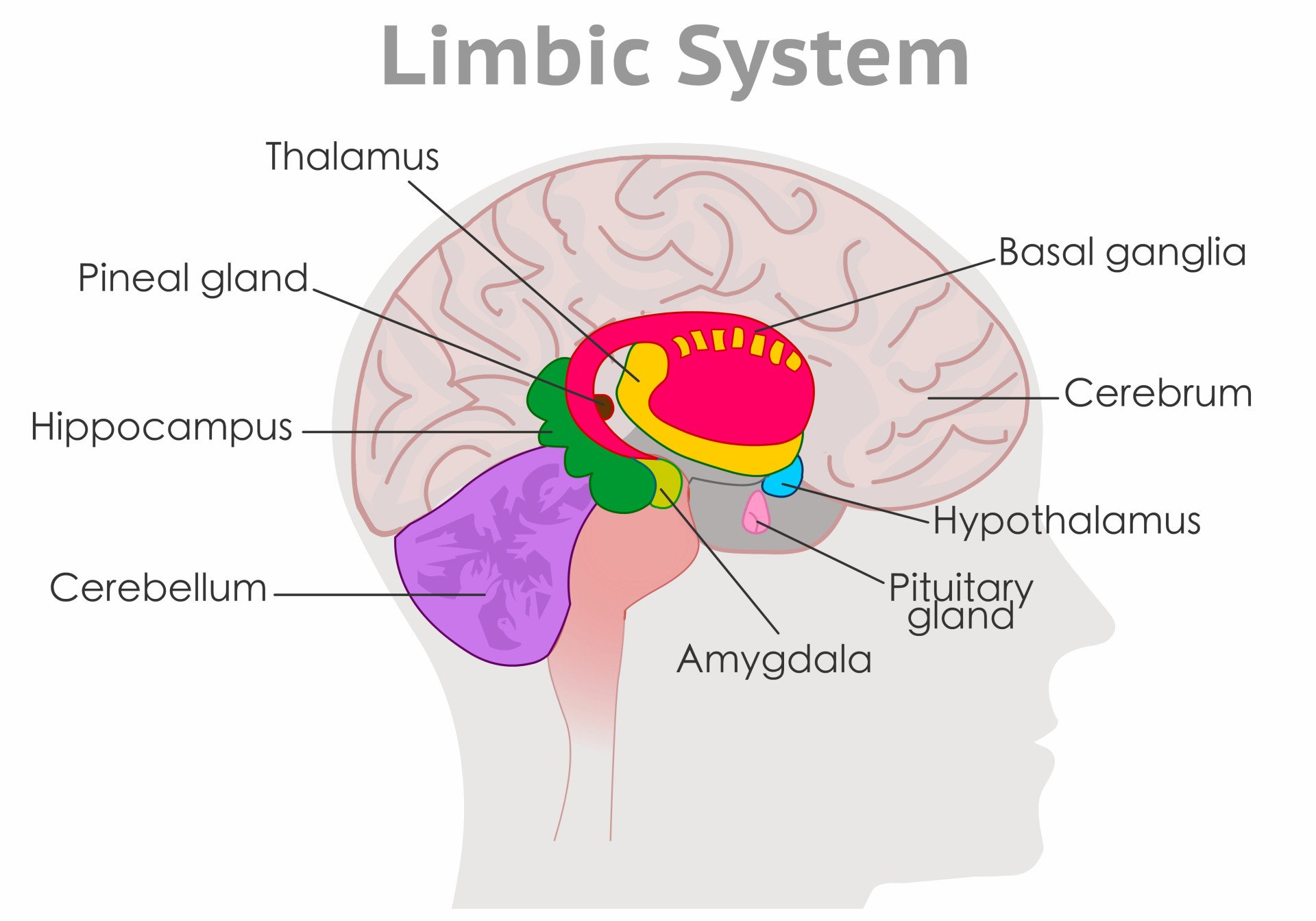
Figure 9. The amygdala in the limbic system plays a key role in how animals assess and respond to environmental threats and challenges by evaluating the emotional importance of sensory information and prompting an appropriate response.
Thalamus and Hypothalamus
The thalamus relays information between the cerebral cortex, brain stem, and other cortical structures (Figure 10).
Because of its interactive role in relaying sensory and motor information, the thalamus contributes to many processes, including attention, perception, timing, and movement. The hypothalamus modulates a range of behavioral and physiological functions.
It controls autonomic functions such as hunger, thirst, body temperature, and sexual activity. To do this, the hypothalamus integrates information from different brain parts and responds to various stimuli such as light, odor, and stress.
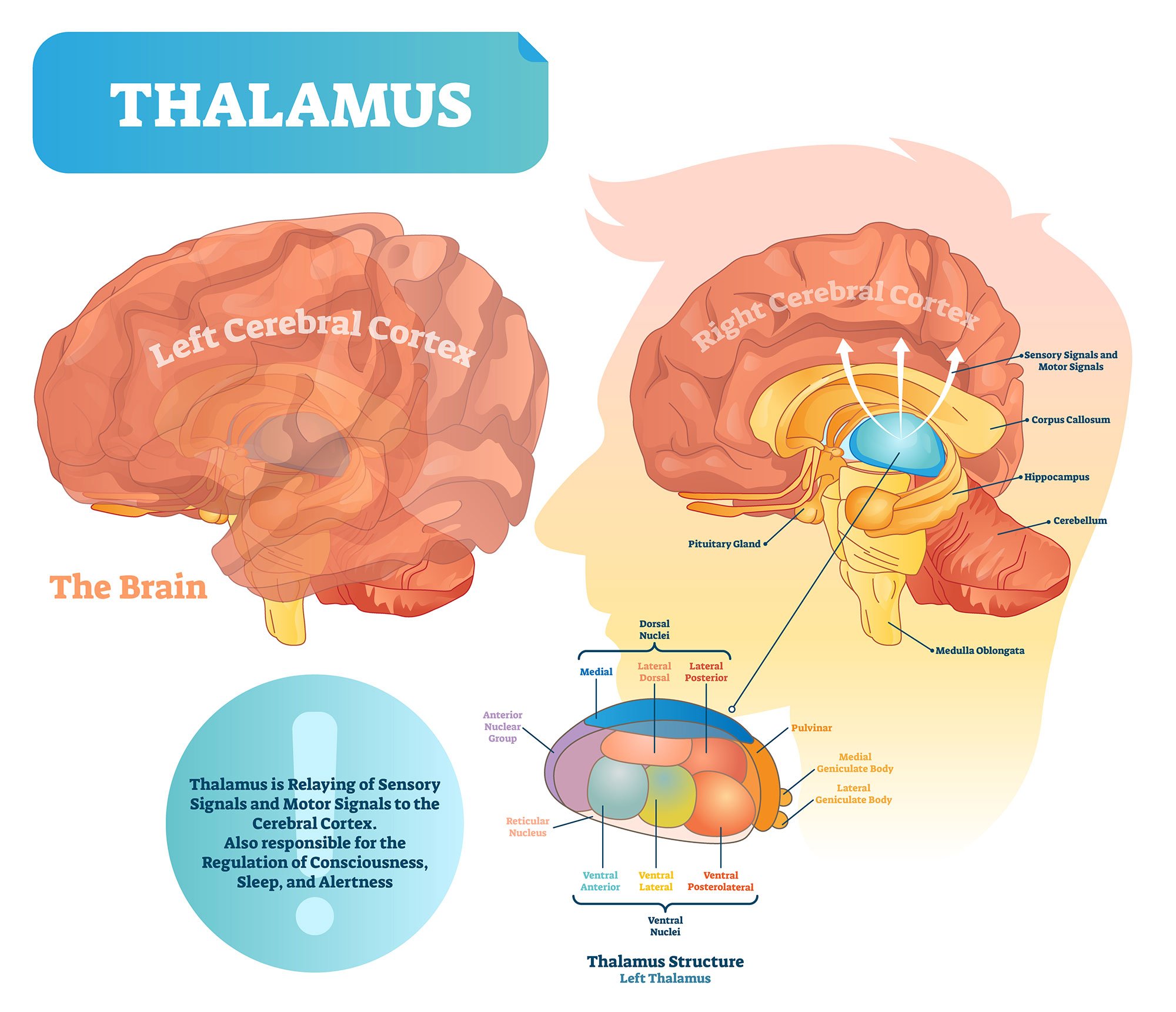
Figure 10. The thalamus is often described as the brain’s relay station as a great deal of information that reaches the cerebral cortex first stops in the thalamus before being sent to its destination.
Hippocampus
The hippocampus is a curved-shaped structure in the limbic system associated with learning and memory (Figure 11).
This structure is most strongly associated with the formation of memories, is an early storage system for new long-term memories, and plays a role in the transition of these long-term memories to more permanent memories.
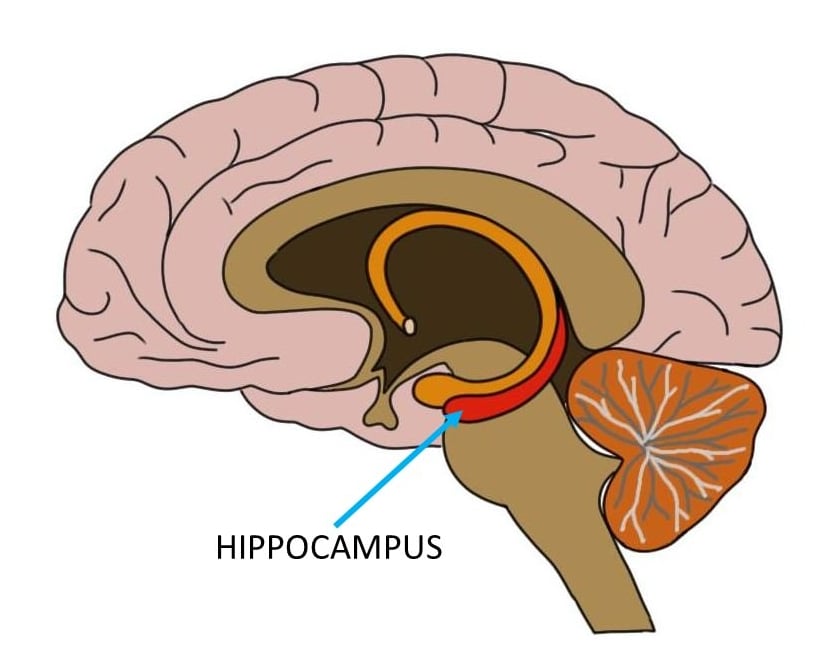
Figure 11. Hippocampus location in the brain
Basal Ganglia
The basal ganglia are a group of structures that regulate the coordination of fine motor movements, balance, and posture alongside the cerebellum.
These structures are connected to other motor areas and link the thalamus with the motor cortex. The basal ganglia are also involved in cognitive and emotional behaviors, as well as playing a role in reward and addiction.
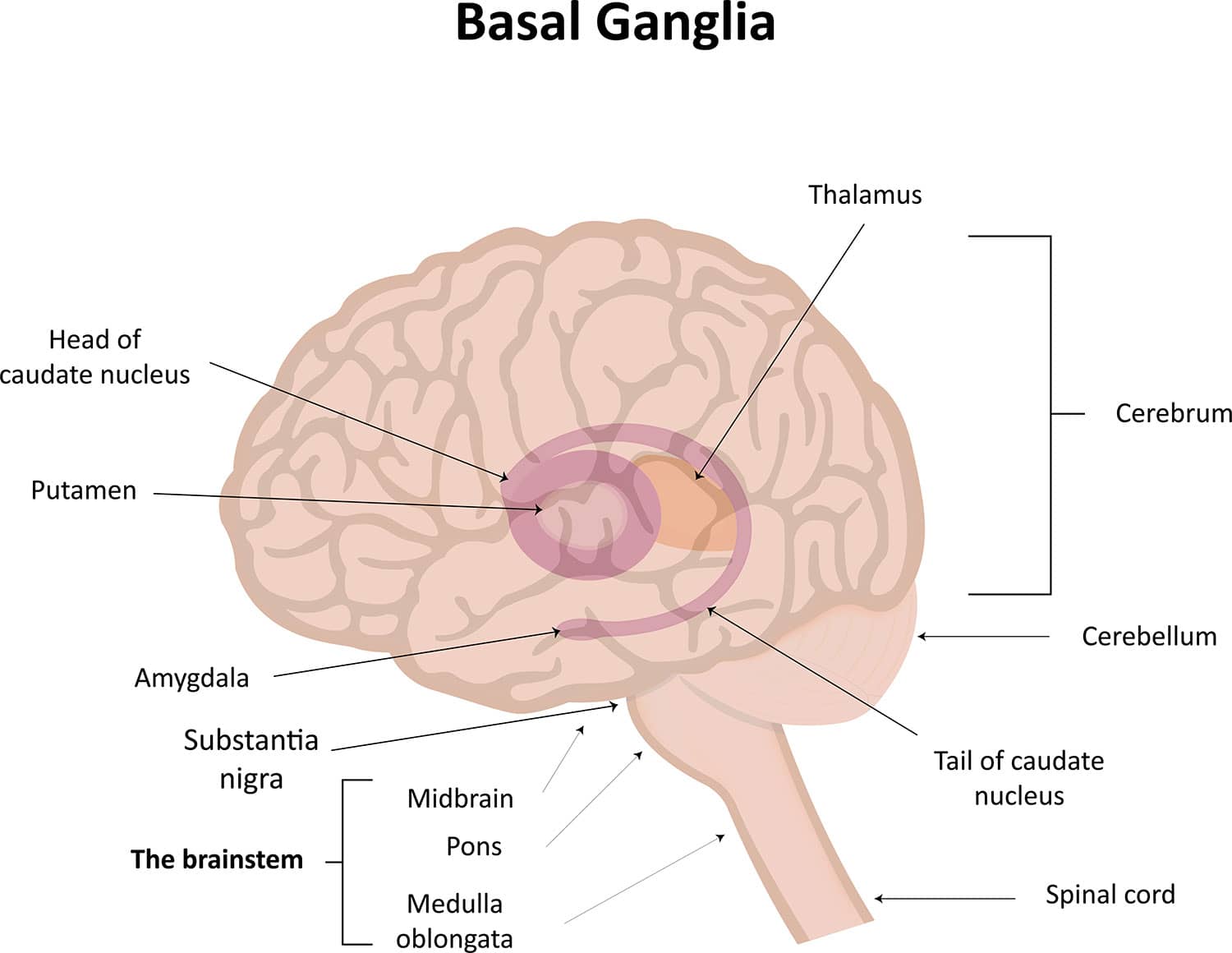
Figure 12. The Basal Ganglia Illustration
Ventricles and Cerebrospinal Fluid
Within the brain, there are fluid-filled interconnected cavities called ventricles , which are extensions of the spinal cord. These are filled with a substance called cerebrospinal fluid, which is a clear and colorless liquid.
The ventricles produce cerebrospinal fluid and transport and remove this fluid. The ventricles do not have a unique function, but they provide cushioning to the brain and are useful for determining the locations of other brain structures.
Cerebrospinal fluid circulates the brain and spinal cord and functions to cushion the brain within the skull. If damage occurs to the skull, the cerebrospinal fluid will act as a shock absorber to help protect the brain from injury.
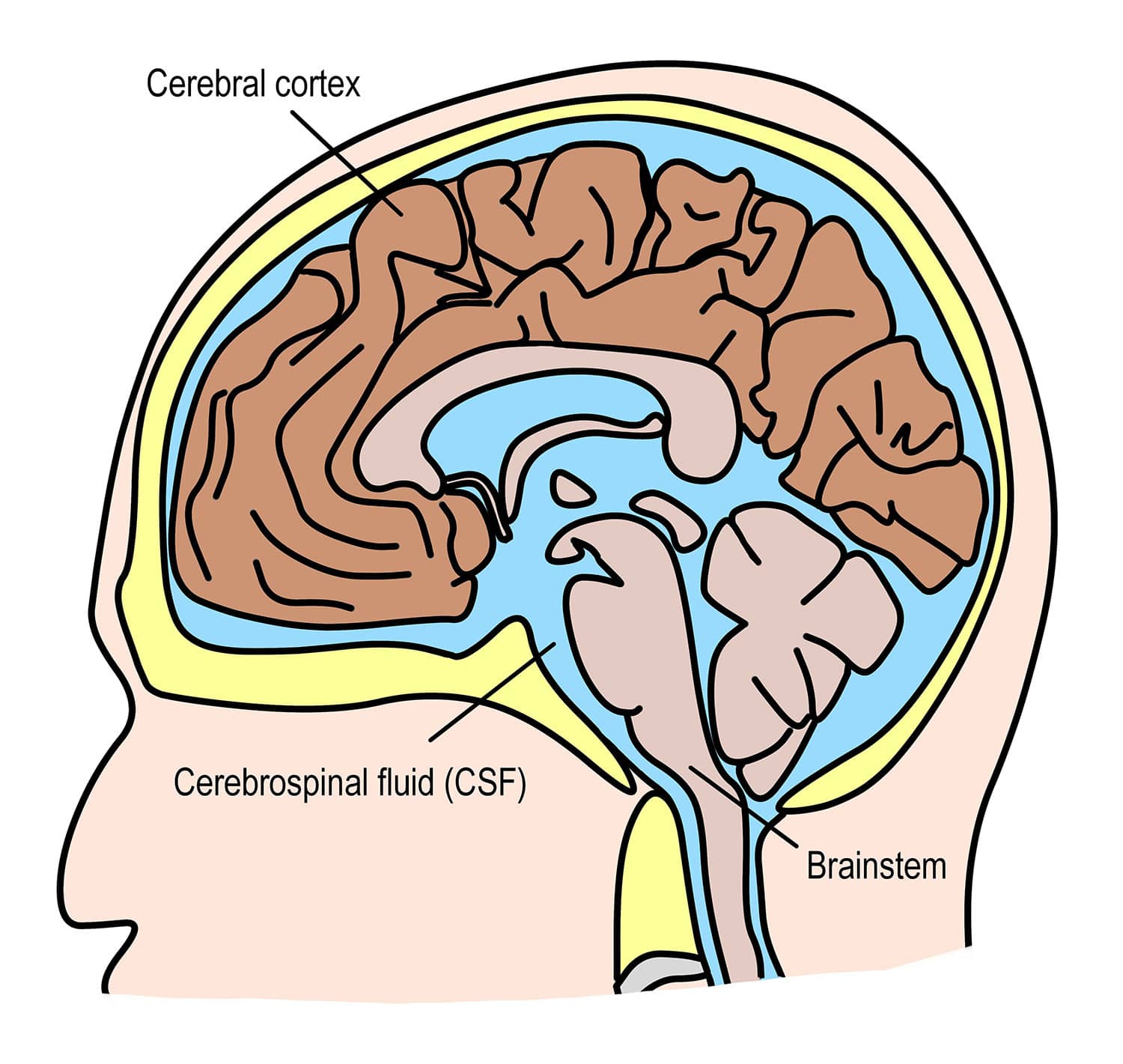
As well as providing cushioning, the cerebrospinal fluid circulates nutrients and chemicals filtered from the blood and removes waste products from the brain. Cerebrospinal fluid is constantly absorbed and replenished by the ventricles.
If there were a disruption or blockage, this can cause a build-up of cerebrospinal fluid and can cause enlarged ventricles.
Neurons are the nerve cells of the central nervous system that transmit information through electrochemical signals throughout the body. Neurons contain a soma, a cell body from which the axon extends.
Axons are nerve fibers that are the longest part of the neuron, which conduct electrical impulses away from the soma.
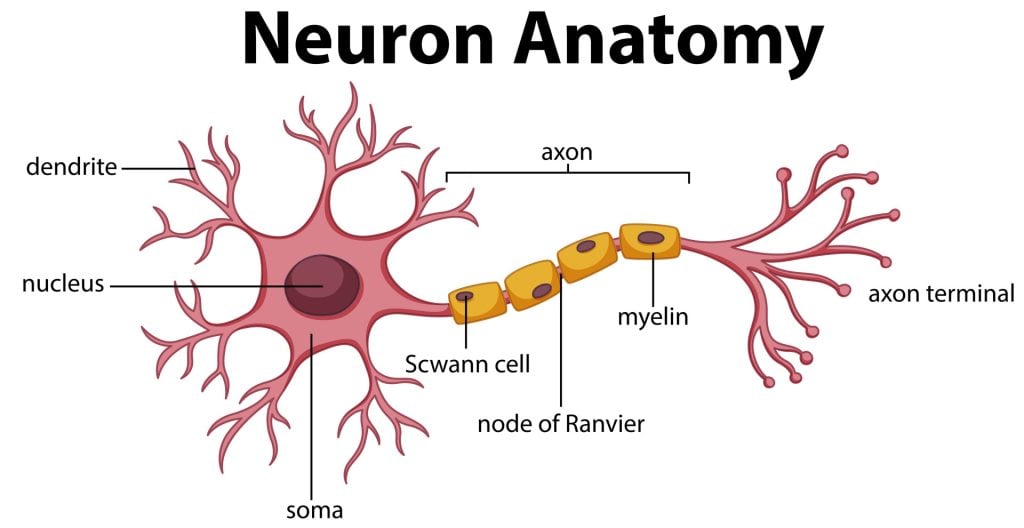
There are dendrites at the end of the neuron, which are branch-like structures that send and receive information from other neurons.
A myelin sheath, a fatty insulating layer, forms around the axon, allowing nerve impulses to travel down the axon quickly.
There are different types of neurons. Sensory neurons transmit sensory information, motor neurons transmit motor information, and relay neurons allow sensory and motor neurons to communicate.
The communication between neurons is called synapses. Neurons communicate with each other via synaptic clefts, which are gaps between the endings of neurons.
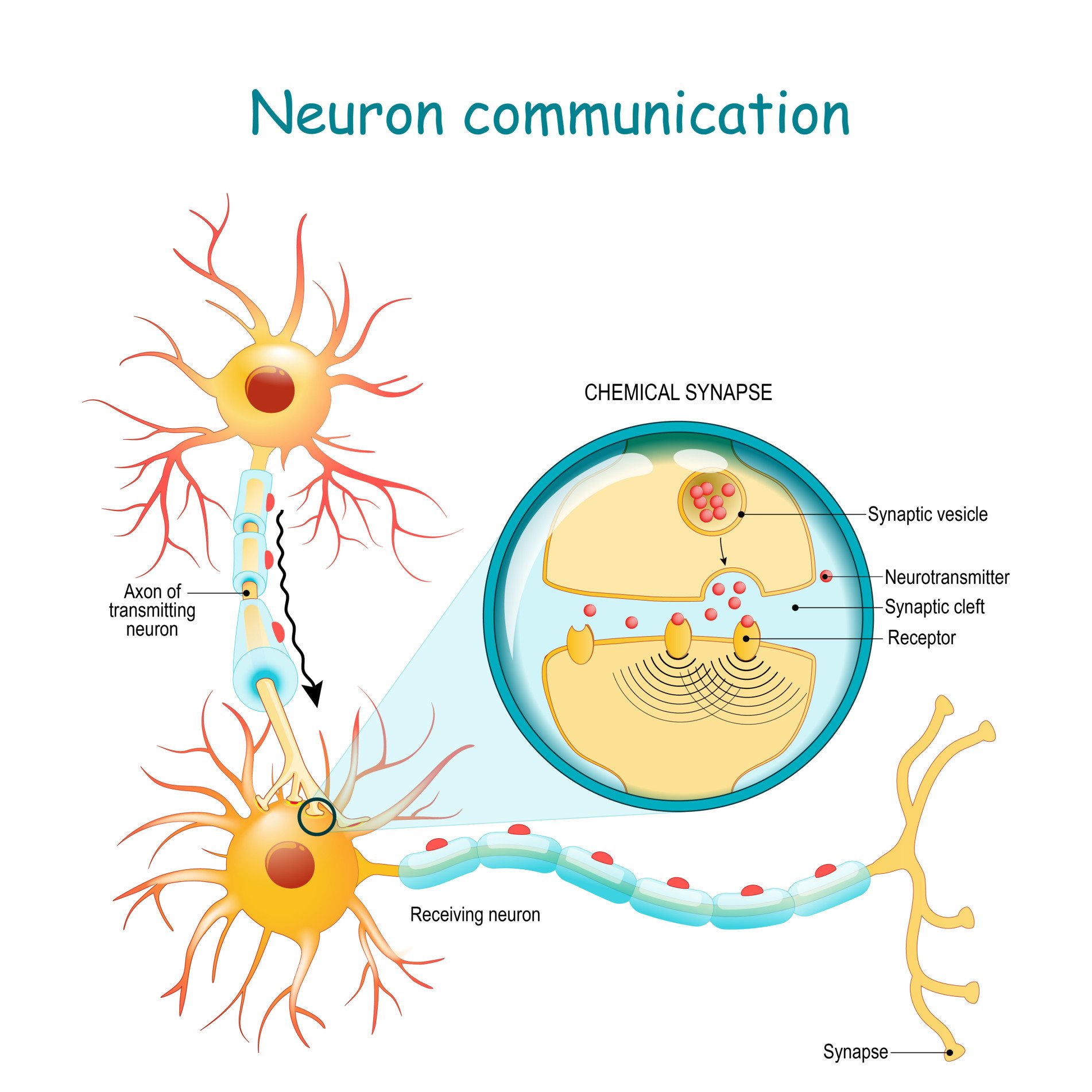
During synaptic transmission, chemicals, such as neurotransmitters, are released from the endings of the previous neuron (also known as the presynaptic neuron).
These chemicals enter the synaptic cleft to then be transported to receptors on the next neuron (also known as the postsynaptic neuron).
Once transported to the next neuron, the chemical messengers continue traveling down neurons to influence many functions, such as behavior and movement.
Glial Cells
Glial cells are non-neuronal cells in the central nervous system which work to provide the neurons with nourishment, support, and protection.
These are star-shaped cells that function to maintain the environment for neuronal signaling by controlling the levels of neurotransmitters surrounding the synapses.
They also work to clean up what is left behind after synaptic transmission, either recycling any leftover neurotransmitters or cleaning up when a neuron dies.
Oligodendrocytes
These types of glial have the appearance of balls with spikes all around them. They function by wrapping around the axons of neurons to form a protective layer called the myelin sheath.
This is a substance that is rich in fat and provides insulation to the neurons to aid neuronal signaling.
Microglial cells have oval bodies and many branches projecting out of them. The primary function of these cells is to respond to injuries or diseases in the central nervous system.
They respond by clearing away any dead cells or removing any harmful toxins or pathogens that may be present, so they are, therefore, important to the brain’s health.
Ependymal cells
These cells are column-shaped and usually line up together to form a membrane called the ependyma. The ependyma is a thin membrane lining the spinal cord and ventricles of the brain .
In the ventricles, these cells have small hairlike structures called cilia, which help encourage the flow of cerebrospinal fluid.
Cranial Nerves
There are 12 types of cranial nerves which are linked directly to the brain without having to pass through the spinal cord. These allow sensory information to pass from the organs of the face to the brain:
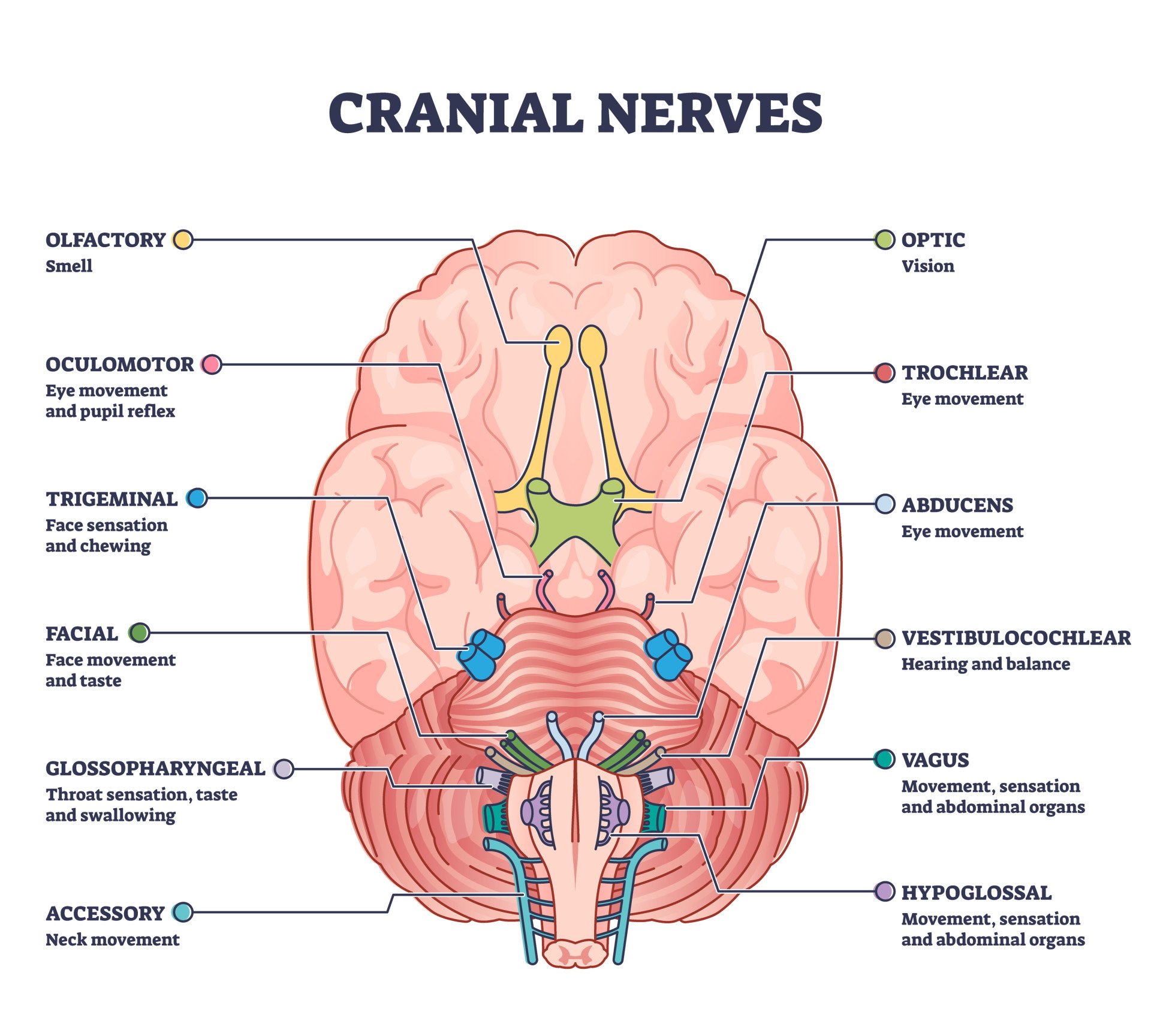
Mnemonic for Order of Cranial Nerves:
S ome S ay M arry M oney B ut M y B rother S ays B ig B rains M atter M ore
- Cranial I: Sensory
- Cranial II: Sensory
- Cranial III: Motor
- Cranial IV: Motor
- Cranial V: Both (sensory & motor)
- Cranial VI: Motor
- Cranial VII: Both (sensory & motor)
- Cranial VIII: Sensory
- Cranial IX: Both (sensory & motor)
- Cranial X: Both (sensory & motor)
- Cranial XI: Motor
- Cranial XII: Motor
Purves, D., Augustine, G., Fitzpatrick, D., Katz, L., LaMantia, A., McNamara, J., & Williams, S. (2001). Neuroscience 2nd edition . sunderland (ma) sinauer associates. Types of Eye Movements and Their Functions.
Mayfield Brain and Spine (n.d.). Anatomy of the Brain. Retrieved July 28, 2021, from: https://mayfieldclinic.com/pe-anatbrain.htm
Robertson, S. (2018, August 23). What is Grey Matter? News Medical Life Sciences. https://www.news-medical.net/health/What-is-Grey-Matter.aspx
Guy-Evans, O. (2021, April 13). Temporal lobe: definition, functions, and location. Simply Psychology. www.www.www.www.www.www.simplypsychology.org/temporal-lobe.html
Guy-Evans, O. (2021, April 15). Parietal lobe: definition, functions, and location. Simply Psychology. www.www.www.www.www.www.simplypsychology.org/parietal-lobe.html
Guy-Evans, O. (2021, April 19). Occipital lobe: definition, functions, and location. Simply Psychology. www.www.www.www.www.www.simplypsychology.org/occipital-lobe.html
Guy-Evans, O. (2021, May 08). Frontal lobe function, location in brain, damage, more. Simply Psychology. www.www.www.www.www.www.simplypsychology.org/frontal-lobe.html
Guy-Evans, O. (2021, June 09). Gyri and sulci of the brain. Simply Psychology. www.www.www.www.www.www.simplypsychology.org/gyri-and-sulci-of-the-brain.html
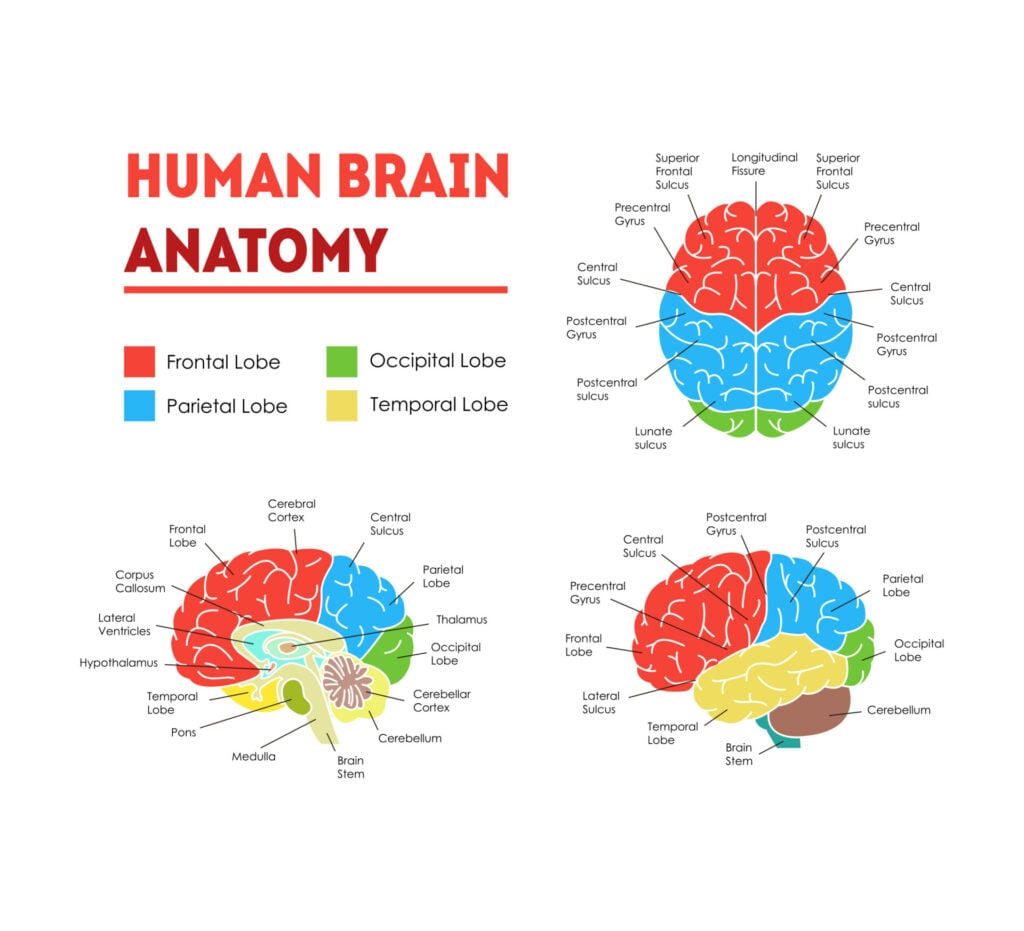
Related Articles
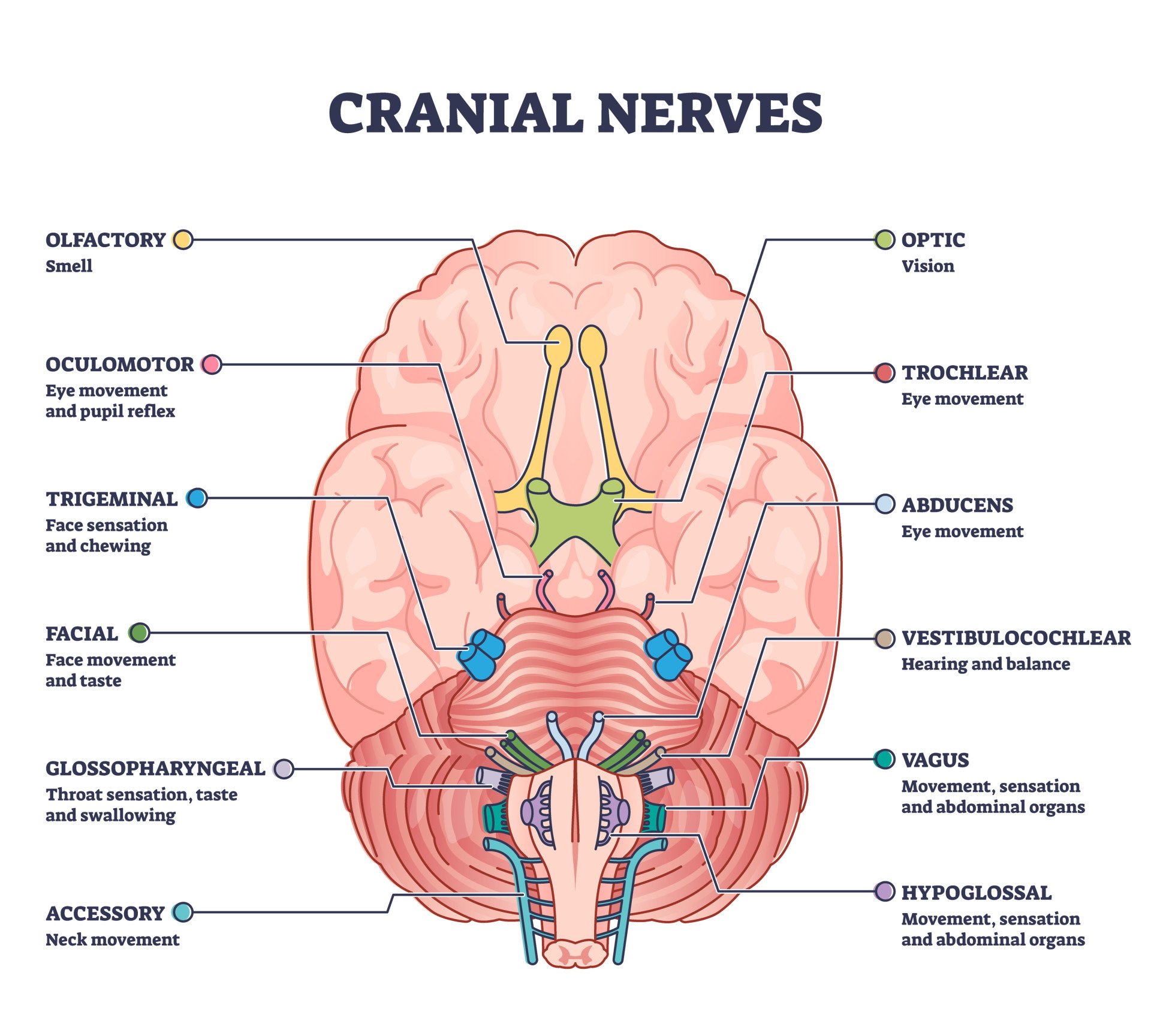
Biopsychology
Summary of the Cranial Nerves
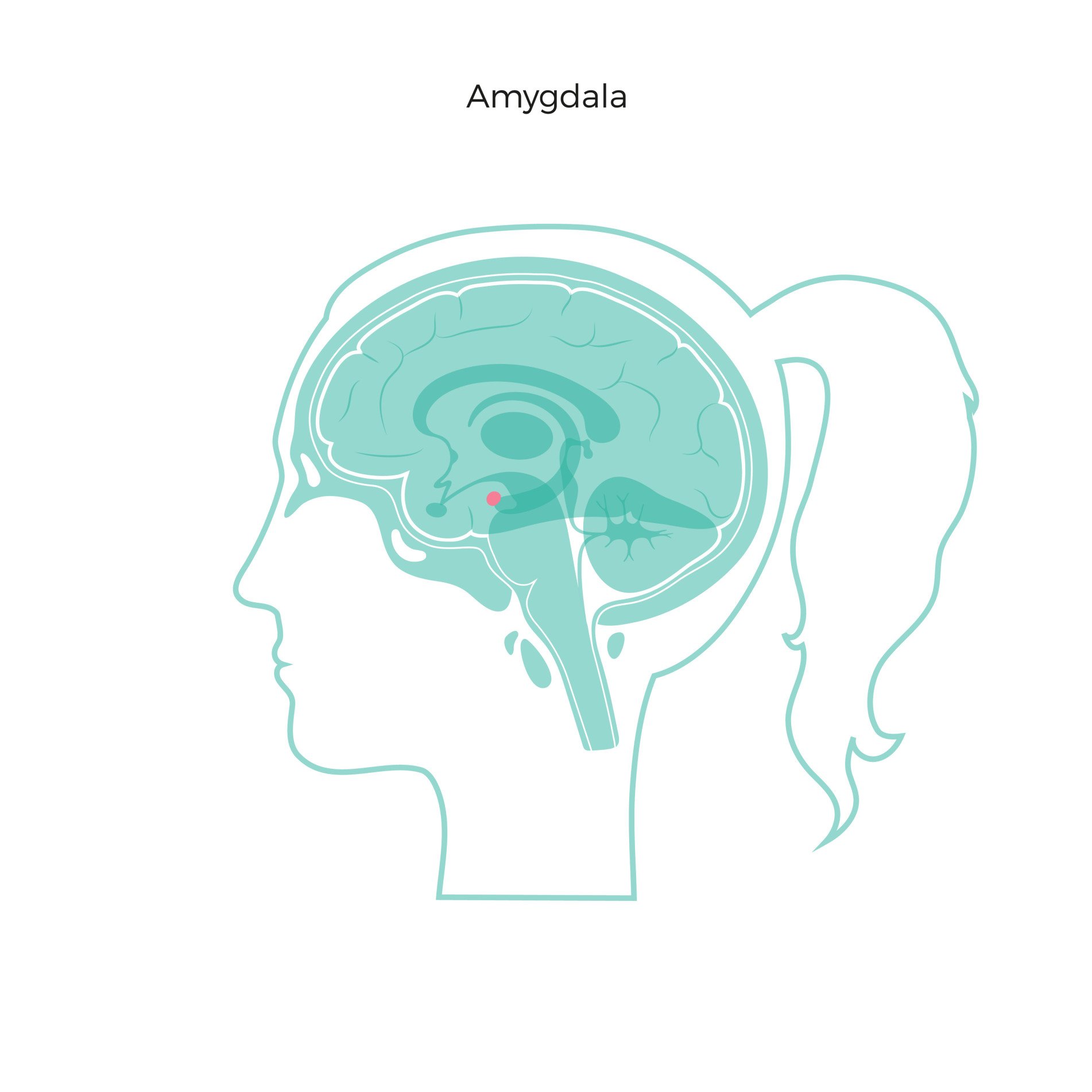
Amygdala: What It Is & Its Functions
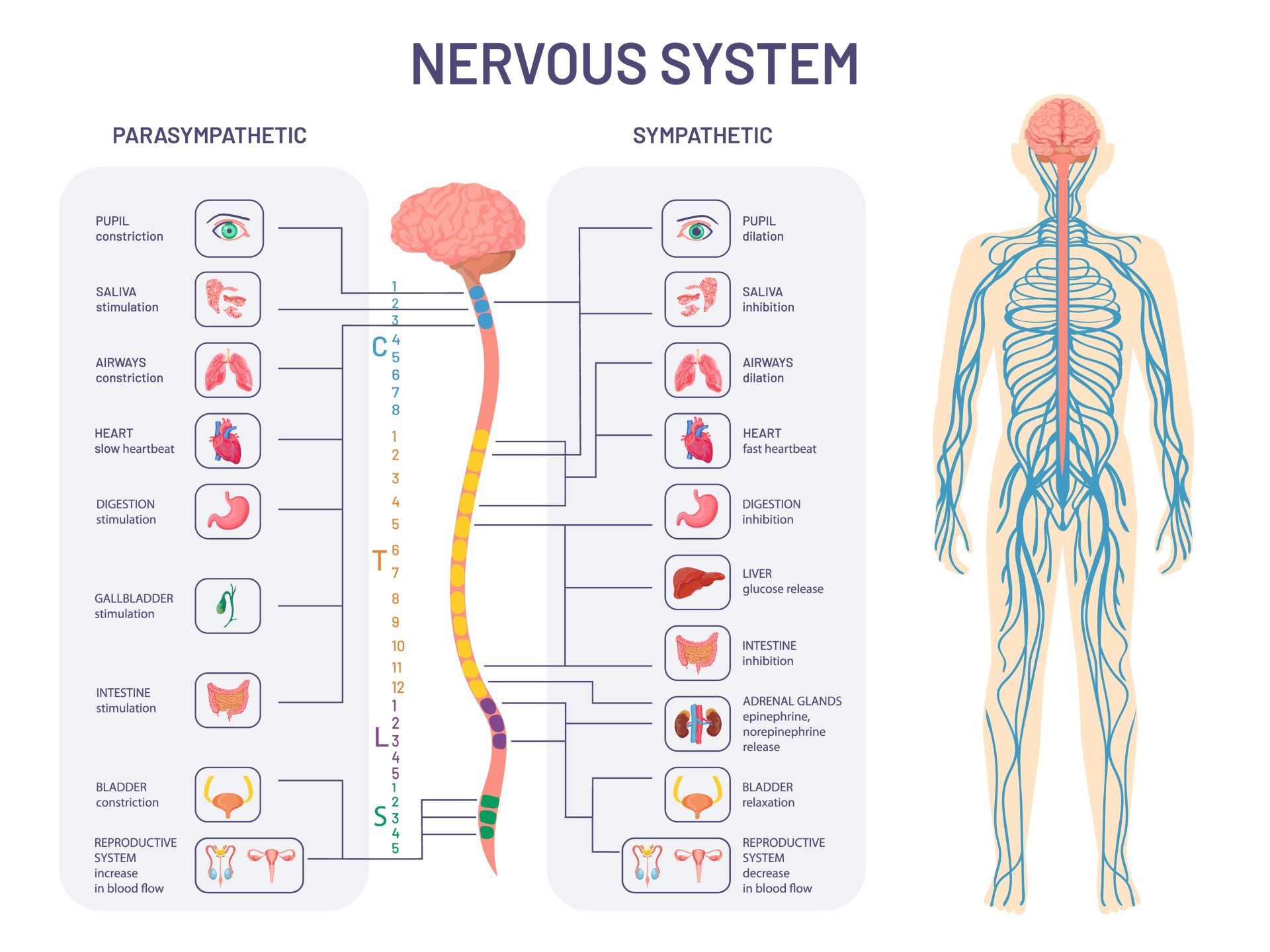
Autonomic Nervous System (ANS): What It Is and How It Works
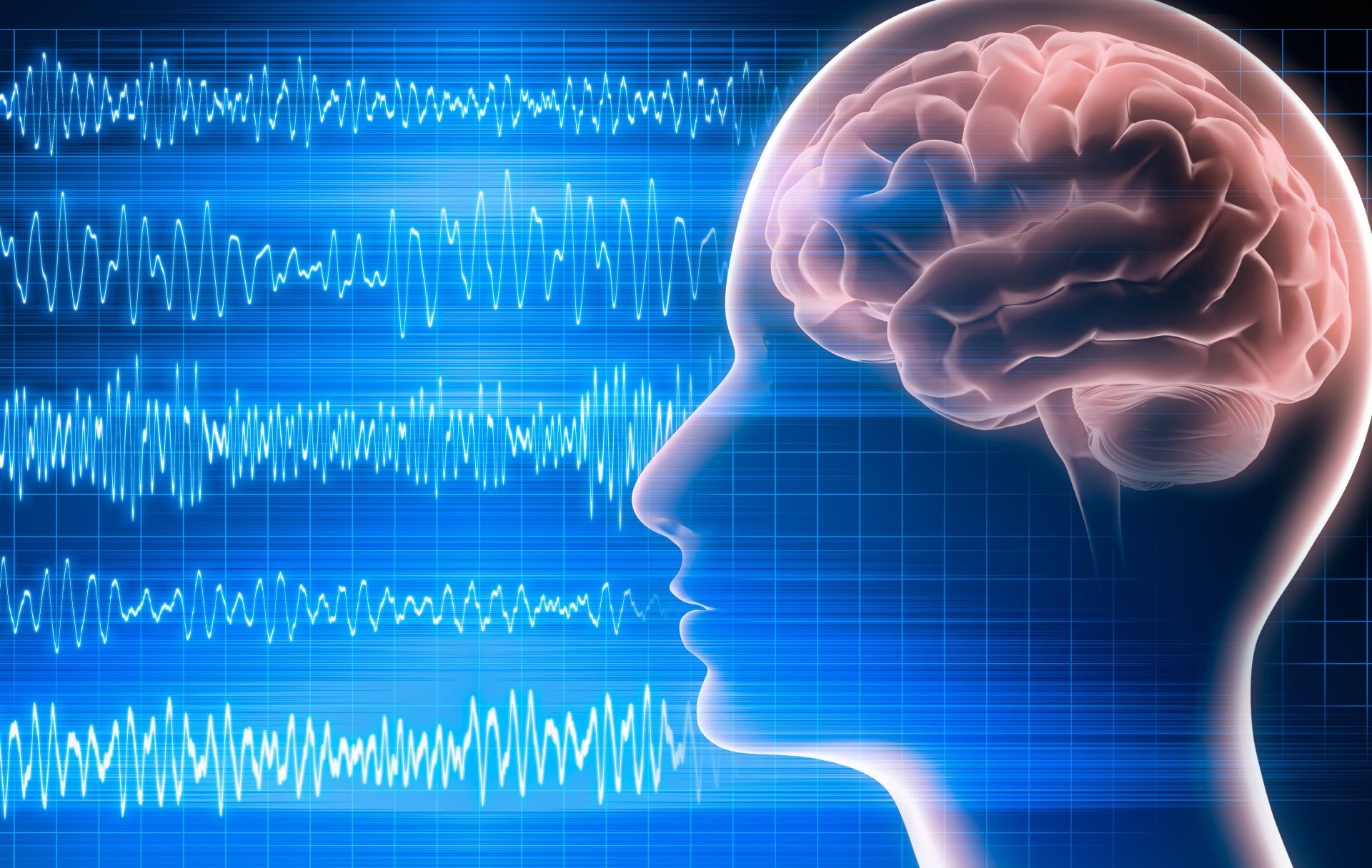
Biopsychology , Psychology
Biological Approach In Psychology
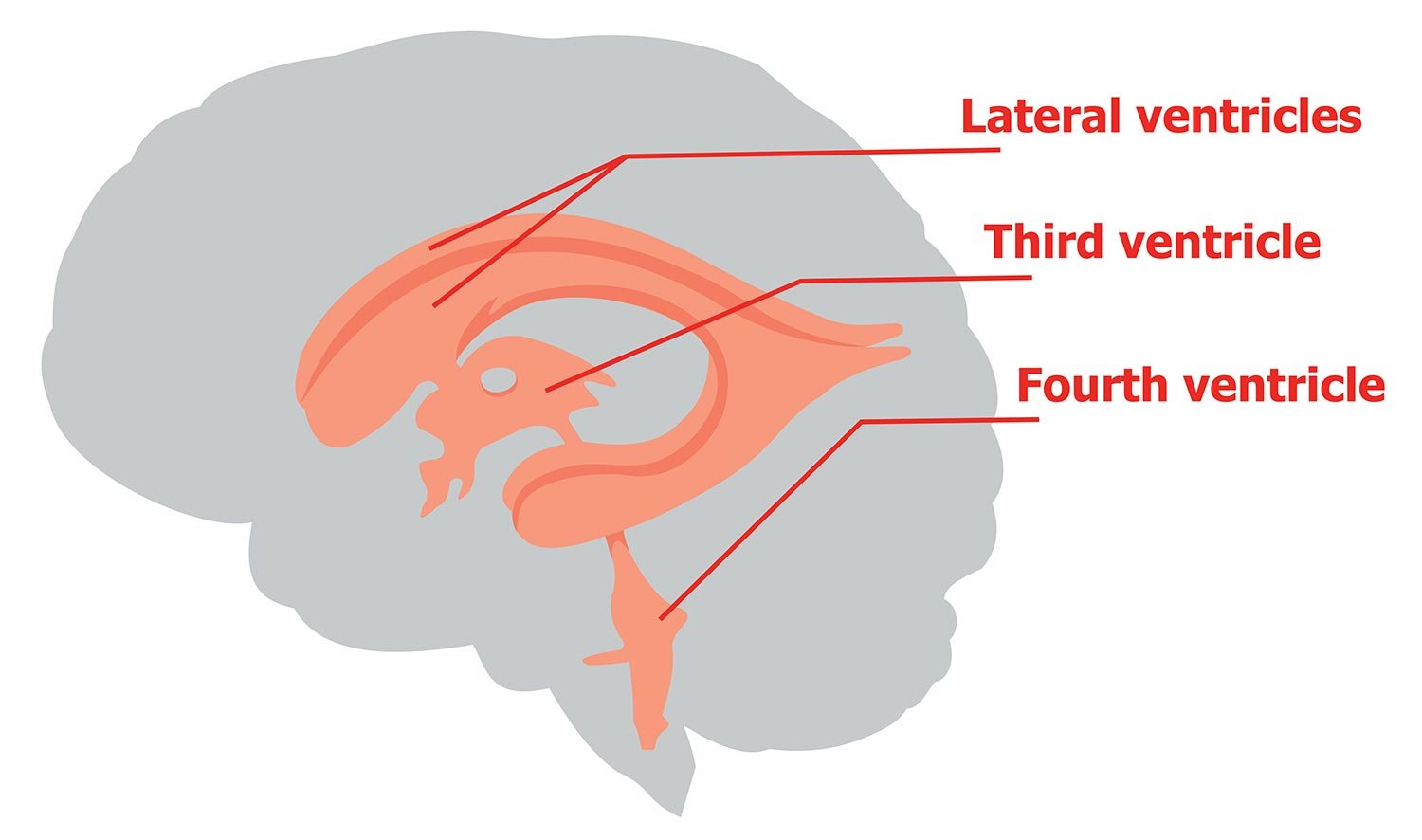
Ventricles of the Brain
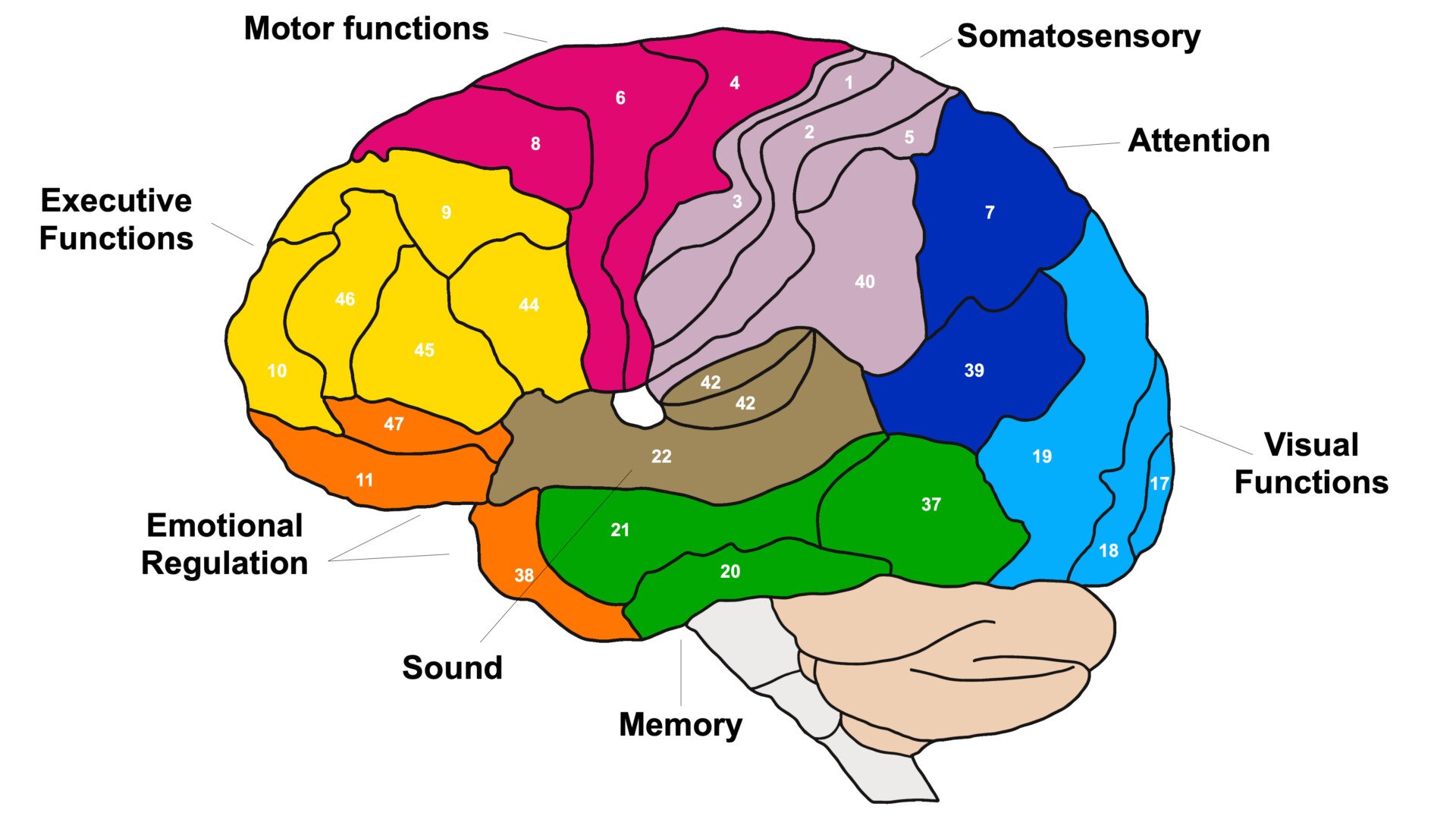
Brodmann Areas Of The Brain

- News/Events
- Arts and Sciences
- Design and the Arts
- Engineering
- Global Futures
- Health Solutions
- Nursing and Health Innovation
- Public Service and Community Solutions
- University College
- Thunderbird School of Global Management
- Polytechnic
- Downtown Phoenix
- Online and Extended
- Lake Havasu
- Research Park
- Washington D.C.
- Biology Bits
- Bird Finder
- Coloring Pages
Experiments and Activities
- Games and Simulations
- Quizzes in Other Languages
- Virtual Reality (VR)
- World of Biology
- Meet Our Biologists
- Listen and Watch
- PLOSable Biology
- All About Autism
- Xs and Ys: How Our Sex Is Decided
- When Blood Types Shouldn’t Mix: Rh and Pregnancy
- What Is the Menstrual Cycle?
- Understanding Intersex
- The Mysterious Case of the Missing Periods
- Summarizing Sex Traits
- Shedding Light on Endometriosis
- Periods: What Should You Expect?
- Menstruation Matters
- Investigating In Vitro Fertilization
- Introducing the IUD
- How Fast Do Embryos Grow?
- Helpful Sex Hormones
- Getting to Know the Germ Layers
- Gender versus Biological Sex: What’s the Difference?
- Gender Identities and Expression
- Focusing on Female Infertility
- Fetal Alcohol Syndrome and Pregnancy
- Ectopic Pregnancy: An Unexpected Path
- Creating Chimeras
- Confronting Human Chimerism
- Cells, Frozen in Time
- EvMed Edits
- Stories in Other Languages
- Virtual Reality
- Zoom Gallery
- Ugly Bug Galleries
- Ask a Question
- Top Questions
- Question Guidelines
- Permissions
- Information Collected
- Author and Artist Notes
- Share Ask A Biologist
- Articles & News
- Our Volunteers
- Teacher Toolbox

show/hide words to know
Disorder: something that is not in order. Not arranged correctly. In medicine a disorder is when something in the body is not working correctly.
Electroencephalogram: visual recording showing the electrical activity of the brain (EEG)... more
Emotion: any of a long list of feelings a person can have such as joy, anger and love... more
What Are the Regions of the Brain and What Do They Do?
The brain has many different parts . The brain also has specific areas that do certain types of work. These areas are called lobes. One lobe works with your eyes when watching a movie. There is a lobe that is controlling your legs and arms when running and kicking a soccer ball. There are two lobes that are involved with reading and writing. Your memories of a favorite event are kept by the same lobe that helps you on a math test. The brain is controlling all of these things and a lot more. Use the map below to take a tour of the regions in the brain and learn what they control in your body.
The brain is a very busy organ. It is the control center for the body. It runs your organs such as your heart and lungs. It is also busy working with other parts of your body. All of your senses – sight, smell, hearing, touch, and taste – depend on your brain. Tasting food with the sensors on your tongue is only possible if the signals from your taste buds are sent to the brain. Once in the brain, the signals are decoded. The sweet flavor of an orange is only sweet if the brain tells you it is.
Brain Waves
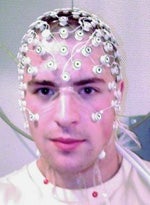
How do you tell if the brain is working? What is it doing and how do you measure it? The head gear on the right that looks like it's from a work of science fiction measures electrical activity in the brain. These electrical waves are called brain waves.
When neurons send a signal they use electrical currents to pass messages to other nearby neurons. Just one or two neurons signaling is too small a change to be noticed. When a huge group of neurons signal at once, however, they can be recorded and measured with the help of special tools.
Measuring electrical activity in the brain is usually done with electrodes. Electrodes are devices able to record electrical changes over time. These are attached to the surface of the skin in specific places around the head. Recordings of brain wave activity look like a series of waves. These are called electroencephalograms, or EEGs for short.
Measuring activity in the brain can be a very useful tool in scientific studies. They can also be used to help identify sleeping disorders and other medical conditions relating to the brain.

The first human electroencephalogram, recorded in 1924 by Hans Berger.
Computer animation credit: BodyParts3D, Copyright© 2010 The Database Center for Life Science licensed under CC Attribution-Share Alike 2.1 Japan.
Read more about: A Nervous Journey
View citation, bibliographic details:.
- Article: What's Your Brain Doing?
- Author(s): Brett Szymik
- Publisher: Arizona State University School of Life Sciences Ask A Biologist
- Site name: ASU - Ask A Biologist
- Date published: May 9, 2011
- Date accessed: May 15, 2024
- Link: https://askabiologist.asu.edu/brain-regions
Brett Szymik. (2011, May 09). What's Your Brain Doing?. ASU - Ask A Biologist. Retrieved May 15, 2024 from https://askabiologist.asu.edu/brain-regions
Chicago Manual of Style
Brett Szymik. "What's Your Brain Doing?". ASU - Ask A Biologist. 09 May, 2011. https://askabiologist.asu.edu/brain-regions
MLA 2017 Style
Brett Szymik. "What's Your Brain Doing?". ASU - Ask A Biologist. 09 May 2011. ASU - Ask A Biologist, Web. 15 May 2024. https://askabiologist.asu.edu/brain-regions
Computer animation image of the human brain. The colors show the frontal lobe (red), parietal lobe (orange), temporal lobe (green), and occipital lobe (yellow).
A Nervous Journey
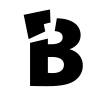
Coloring Pages and Worksheets
Neuron Anatomy
What's In Your Brain
What's Your Brain Doing?

Be Part of Ask A Biologist
By volunteering, or simply sending us feedback on the site. Scientists, teachers, writers, illustrators, and translators are all important to the program. If you are interested in helping with the website we have a Volunteers page to get the process started.
Share to Google Classroom
Areas of the Brain
Reviewed by Psychology Today Staff
The human brain, as the seat of mental life—from the most complicated intellectual processes down to routine and unconscious bodily control—is necessarily enormously complex.
The largest part of the brain is the highly-evolved cerebrum. The topmost portion is divided into two hemispheres, each with four lobes, within which are many other subdivisions. The cerebral cortex forms the outer layer of the cerebrum. Nested deeper within the brain are essential structures such as the basal ganglia, the amygdala, and the hippocampus. Toward the bottom of the brain are more primitive structures, including the cerebellum and brain stem. These also fulfill critical functions.
Running through parts of the brain are a dozen pairs of cranial nerves, which link the brain directly to muscles and sense organs in the head, neck, and upper body. The brain also contains four interconnected spaces called ventricles, which produce and are filled with cerebrospinal fluid. This fluid is distributed to the exterior of the brain and to the spinal cord, where it provides a cushion against physical impact and helps maintain normal function.
The brain and spinal cord together form the body’s command structure: the central nervous system. The peripheral nervous system extends body-wide, encompassing nerves that branch out from the spinal cord and the brain.
On This Page
- Hemispheres of the Brain
- Lobes of the Brain
- Beneath the Cortex
The cerebrum is physically divided into two halves—the left hemisphere and right hemisphere—and the functions of which are specialized in certain ways. Both hemispheres work closely together , communicating via a bundle of nerve fibers, the corpus callosum, that forms a bridge between them.
The left hemisphere controls the movement of the opposite (right) side of the body. Among the tasks for which it is specialized are the ability to speak and aspects of language comprehension, though language ability involves both hemispheres. Broca’s area and Wernicke’s area, parts of the brain that are both linked to language, are typically located in the left hemisphere.
The right hemisphere controls the movement of the left side of the body. The right hemisphere is specialized relative to the left in some aspects of spatial processing, including that of three-dimensional relations between objects. It also appears to be especially important for processing non-literal aspects of language, such as metaphors.
While each hemisphere has certain tasks to which it is especially tailored, broad mental activities and qualities such as perception, creativity, and reasoning are handled by both hemispheres together. (For more, see Left Brain - Right Brain. )
![Wikimedia Commons [Public Domain] Wikimedia Commons [Public Domain]](https://cdn2.psychologytoday.com/assets/styles/manual_crop_1_1_180x180/public/2021-01/brain%20lobes.jpg?itok=YUplVOIZ)
The cerebral hemispheres are commonly mapped out based on four large regions—the frontal lobe, the temporal lobe, the parietal lobe, and the occipital lobe—with one of each on either half of the brain.
- The frontal lobes form the front of the cerebrum, starting directly behind the forehead. Important for executive functioning, the frontal lobes are involved in decision-making, attention, producing voluntary movements (controlled by the motor area of the frontal lobe), and speech, along with various other voluntary and involuntary abilities.
- The parietal lobes are located behind the frontal lobes. The parietal lobes contain the primary sensory areas, which are involved in processing information about taste, touch, temperature, and movement. The parietal lobes also play a role in language processing, including reading.
- The temporal lobes sit on the sides of the brain. They contain the primary auditory cortices and process information from the ears; they also work with the occipital lobes to process visual information. The temporal lobes are heavily involved in learning and memory. The medial temporal lobe contains important structures including the hippocampus and amygdala.
- The occipital lobes form the back section of the cerebrum. They contain the primary visual cortices and are crucial for vision.
The cerebral cortex is the thin, outermost layer of the cerebrum, extending across all the brain’s lobes. Just 1.5 to 5 millimeters in width, it is composed of the neurons that make up the brain’s “gray matter” and handles much of the brain’s work. (The gray matter of the cortex is interlinked with the rest of the brain by nerve fibers that make up the brain’s “white matter.”)
The cortex’s distinctive, wrinkled appearance is due to its various ridges (gyri) and grooves (sulci). These provide for a larger total surface area within a condensed space—and hence more room for neurons—than would a totally smooth layer of brain cells.
Buried in the core of the brain are a collection of structures that serve a variety of important functions. Some of these essential components are:
- The thalamus is akin to a relay station, receiving signals going to and from the cerebrum and other parts of the nervous system. It is an integral part of the system of sensory processing, but is also involved in movement, emotion, memory, and other functions. The brain contains two thalami, one on either side.
- The roughly pea-sized hypothalamus , below the thalamus, plays a role in bodily regulation, including the control of body temperature, sleep and wakefulness, hunger, and the release of the stress hormone cortisol and other hormones. The hypothalamus produces a number of hormones itself, including oxytocin, dopamine, vasopressin, and others. It is directly connected to and influences the functioning of the pituitary gland, which releases various hormones into the bloodstream.
- The amygdala is an almond-shaped structure that has a major role in producing emotional responses. It also helps to integrate emotional aspects of experience into memory and to produce fear-based memories and learning. There are a pair of amygdalae in the brain. The amygdala and its neighbor the hippocampus are two major components of the limbic system.
- The hippocampus , named for its resemblance of a seahorse's shape, is central to the creation and filing-away of memories. It is also involved in spatial processing and the ability to find one’s way around. There are two hippocampi in the brain. Along with the amygdalae, they are major parts of the limbic system.
- The clusters of neurons called the basal ganglia are important for coordinating physical movements, including habitual behaviors.
The cerebellum (Latin for “little brain”) is packed with 50 percent of the neurons contained in the whole central nervous system. It is located at the rear and toward the bottom of the brain, beneath the cerebrum and behind the brainstem. Like the larger cerebrum, it contains two hemispheres of its own, each containing an outer layer of grey matter and an inner area of white matter.
The cerebellum plays a central role in the control of the body’s movements and in the learning of physical tasks. Based on signals from the inner ears and the muscles, the cerebellum enables the body to maintain balance and posture.
The brain stem, situated at the bottom of the brain, is made up of three main parts: the midbrain, pons, and medulla oblongata.
- The midbrain is the highest part of the brain stem. Among other functions, it is responsible for certain reflexes, helps to process visual and auditory information, and contributes to the control of eye movement as well as other body movements.
- The pons (from the Latin for “bridge”) is a base for connections between different parts of the brain, including between the cerebellum and the cerebral cortex as well as between the cerebellum and the medulla. It contains the endpoints of several cranial nerves that link the face region and the brain.
- The medulla oblongata (or medulla) is the lowest part of the brain stem. It is important for the control of basic, involuntary functions including breathing, digestion, heart rate, and certain reflexes (such as the gag reflex and sneezing). It is directly joined with the spinal cord and helps to carry signals from the spine to the rest of the brain.
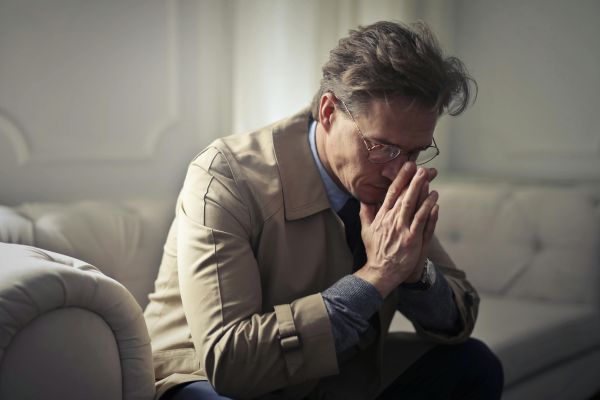
About one out of ten people is left-handed but the reasons why are not well understood. A new study now helps unlock the mystery of left-handedness.
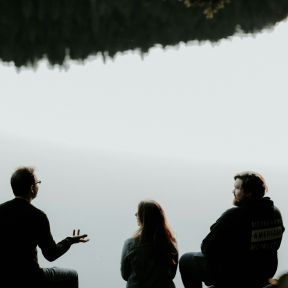
What enables effective, accurate communication? It comes down to the similarity of two brains during communication, a phenomenon known as neural coupling.
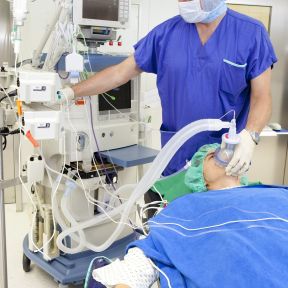
An abundance of evidence supports the relationship between anesthesia and memory loss. The severity and frequency of exposure to anesthesia could lead to mild cognitive impairment.
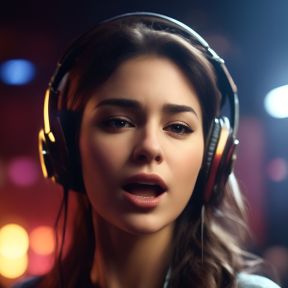
Music-induced pleasure relies on the engagement of both higher-order brain regions as well as some primitive reward-related areas.
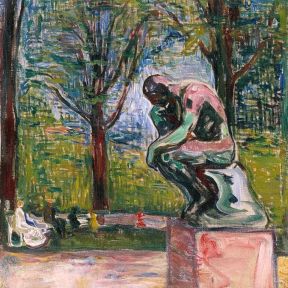
That little voice inside your head? The puzzle of consciousness would still be there without it. Here's why.
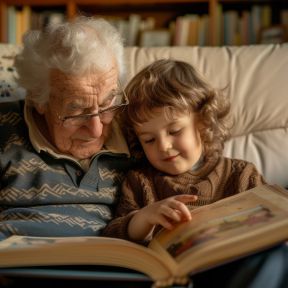
Is it better for kids to read books or spend time on screens? Research shows early reading for pleasure is a game-changer for healthy brain development. Screen time? Not so much.
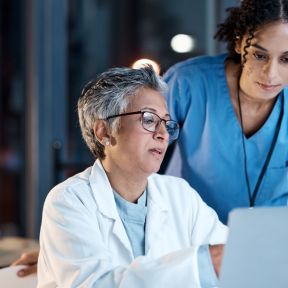
The time is now for the AI community to build explicit reasoning systems in service of implicit reasoning LLMs to maintain momentum in healthcare advances.
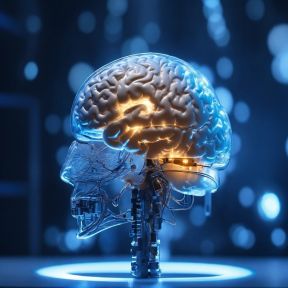
AI deep learning powers a brain-computer interface that enables humans to continuously control a cursor using thoughts.
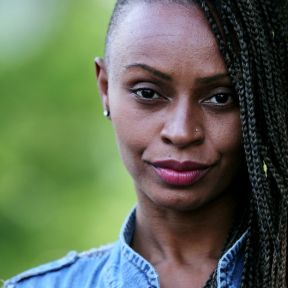
Left-brained? Right-brained? No, just...brained.
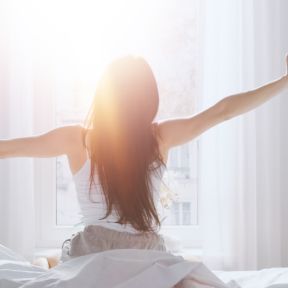
Sleeping in leads to cascades of negative effects that can interfere with cardiac and metabolic health and body weight regulation.
- Find a Therapist
- Find a Treatment Center
- Find a Psychiatrist
- Find a Support Group
- Find Online Therapy
- United States
- Brooklyn, NY
- Chicago, IL
- Houston, TX
- Los Angeles, CA
- New York, NY
- Portland, OR
- San Diego, CA
- San Francisco, CA
- Seattle, WA
- Washington, DC
- Asperger's
- Bipolar Disorder
- Chronic Pain
- Eating Disorders
- Passive Aggression
- Personality
- Goal Setting
- Positive Psychology
- Stopping Smoking
- Low Sexual Desire
- Relationships
- Child Development
- Self Tests NEW
- Therapy Center
- Diagnosis Dictionary
- Types of Therapy
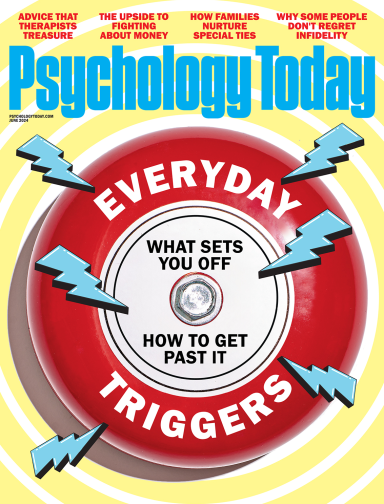
At any moment, someone’s aggravating behavior or our own bad luck can set us off on an emotional spiral that threatens to derail our entire day. Here’s how we can face our triggers with less reactivity so that we can get on with our lives.
- Emotional Intelligence
- Gaslighting
- Affective Forecasting
- Neuroscience
Brain Dysfunction by Location
- Frontal Lobe Damage |
- Parietal Lobe Damage |
- Temporal Lobe Damage |
- Occipital Lobe Damage |
- Limbic Lobe Damage |
- Other Locations |
Because different areas of the brain control specific functions, the location of brain damage determines the type of dysfunction that results.
Parts of the Brain
Which side of the brain is affected is also important because the functions of the two halves of the cerebrum (cerebral hemispheres) are not identical. Some functions of the brain are performed exclusively by one hemisphere. For example, movement and sensation on one side of the body are controlled by the hemisphere on the opposite side. Other functions are performed mainly by one hemisphere, which is said to be dominant for that function, and the other hemisphere is said to be nondominant. For example, the left hemisphere mainly controls language in most people. This characteristic is called left-hemisphere language dominance. Damage to only one hemisphere of the brain may cause complete loss of such functions.
However, most functions (such as memory) require coordination of several areas in both hemispheres. For such functions to be completely lost, both hemispheres must be damaged.
Specific patterns of dysfunction can be related to the area of the brain that has been damaged.
Usually, doctors can diagnose the type of dysfunction by examining the person. They ask questions designed to evaluate specific brain functions. Imaging tests, such as computed tomography (CT) and magnetic resonance imaging (MRI), are usually needed to identify the cause of the damage.
Frontal Lobe Damage
The frontal lobes have the following functions:
Initiating many actions
Controlling learned motor skills, such as writing, playing musical instruments, and tying shoelaces
Controlling complex intellectual processes, such as speech, thought, concentration, problem-solving, and planning for the future
Controlling facial expressions and hand and arm gestures
Coordinating expressions and gestures with mood and feelings
Generally, damage to the frontal lobes causes loss of the ability to solve problems and to plan and initiate actions, such as crossing the street or answering a complex question (sometimes called executive functions). But some specific impairments vary depending on which part of the frontal lobe is damaged.
If the back part of the frontal lobe (which controls voluntary movements) is damaged, weakness or paralysis can result. Because each side of the brain controls movement of the opposite side of the body, damage to the left hemisphere causes weakness on the right side of the body, and vice versa.
If the middle part of the frontal lobe is damaged, people may become apathetic, inattentive, and unmotivated. Their thinking becomes slow, and their responses to questions are very slow.
If the middle back part of the left frontal lobe (Broca area) is damaged, people may have difficulty expressing themselves in words—an impairment called Broca (expressive) aphasia .
If the front part of the frontal lobe is damaged, any of the following may result:
Difficulty temporarily holding information available for processing (called working memory)
Reduced fluency of speech
Apathy (lack of emotion, interest, and concern)
Inattentiveness
Delayed responses to questions
A striking lack of inhibition, including socially inappropriate behavior
People who lose their inhibitions may be inappropriately elated (euphoric) or depressed, excessively argumentative or passive, and vulgar. They may show no regard for the consequences of their behavior. They may also repeat what they say. Some people develop similar symptoms when they get older or if dementia develops. These symptoms may result from degeneration of the frontal lobe.
When Specific Areas of the Brain Are Damaged
Parietal lobe damage.
The parietal lobes have the following functions:
Interpreting sensory information from the rest of the body
Combining impressions of form, texture, and weight into general perceptions
Influencing mathematical skills and language comprehension
Storing spatial memories that enable people to orient themselves in space (know where they are) and to maintain a sense of direction (know where they are going)
Processing information that helps people know the position of their body parts
Certain functions tend to be controlled more by one of the parietal lobes (usually the left). It is considered the dominant lobe when it controls language. The other lobe (nondominant) has other functions, such as enabling people to be aware of how the body relates to the space around it.
Damage to the front part of the parietal lobe on one side causes numbness and impairs sensation on the opposite side of the body. Affected people have difficulty identifying a sensation’s location and type (pain, heat, cold, or vibration). People may have difficulty recognizing objects by touch (that is, by their texture and shape).
If the middle part is damaged, people cannot tell the right from the left side (called right-left disorientation) and have problems with calculations and writing. They may have problems sensing where parts of their body are (a sense called proprioception).
If the nondominant (usually right) parietal lobe is damaged, people may be unable to do simple skilled tasks, such as combing their hair or dressing—called apraxia . They may also have trouble understanding how objects relate to each other in space. As a result, they may have trouble drawing and constructing things, and they may get lost in their own neighborhood. These people may also ignore the serious nature of their disorder or deny its existence. They may neglect the side of the body opposite the brain damage (usually the left side).
Temporal Lobe Damage
The temporal lobes have the following functions:
Generating memory and emotions
Processing immediate events into recent and long-term memory
Storing and retrieving long-term memories
Comprehending sounds and images, enabling people to recognize other people and objects and to integrate hearing and speech
In most people, part of the left temporal lobe controls language comprehension. If that part is damaged, memory for words can be drastically impaired, as can the ability to understand language—an impairment called Wernicke (receptive) aphasia (see table Testing a Person With Aphasia ).
If certain areas of the right temporal lobe are damaged, memory for sounds and music may be impaired. As a result, people may have trouble singing.
Occipital Lobe Damage
The occipital lobe contains the main center for processing visual information.
The occipital lobes have the following functions:
Processing and interpreting vision
Enabling people to form visual memories
Integrating visual perceptions with the spatial information provided by the adjacent parietal lobes
If both sides of the occipital lobe are damaged, people cannot recognize objects by sight, even though the eyes themselves are functioning normally. This disorder is called cortical blindness. Some people with cortical blindness are unaware that they cannot see. Instead, they often make up descriptions of what they see (called confabulation). This disorder is called Anton syndrome.
Seizures that involve the occipital lobe can cause hallucinations involving vision. For example, people may see lines of color when they look in a certain direction.
Limbic Lobe Damage
The limbic lobe includes structures located deep within the cerebrum and some parts of the adjacent lobes, such as the temporal lobe. These structures have the following functions:
Receiving and integrating information from many areas of the brain, enabling people to experience and express emotions
Helping form and retrieve memories
Helping people connect memories to the emotions experienced when the memories form
Damage that affects the limbic lobe usually results in a variety of problems.
Seizures that result from damage to the temporal lobe area in the limbic lobe usually last only a few minutes. At first, people may not be able to control their feelings or to think clearly. Or they may smell bad odors that are not there (a type of hallucination). They may appear dazed and unaware of their surroundings and make automatic movements, such as repeatedly swallowing or smacking their lips. During the seizure, some people have personality changes such as humorlessness, extreme religiosity, and obsessiveness. People may also have an overwhelming urge to write.
Other Locations
Many functions of the brain are performed by several areas of the brain working together (networks), not by a single area in the brain. Damage to these networks can cause the following:
Agnosia (loss of the ability to identify objects using one or more of the senses)
Amnesia (total or partial loss of the ability to recall experiences or events)
Aphasia (partial or complete loss of the ability to express or understand spoken or written language)
Apraxia (loss of the ability to do tasks that require remembering patterns or sequences of movements)
Dysarthria (loss of the ability to articulate words normally) may be caused by damage to areas of the brain or cranial nerves that control the muscles involved in producing speech or by damage to the nerve fibers that connect these areas.

- Cookie Preferences

Copyright © 2024 Merck & Co., Inc., Rahway, NJ, USA and its affiliates. All rights reserved.

An official website of the United States government
The .gov means it's official. Federal government websites often end in .gov or .mil. Before sharing sensitive information, make sure you're on a federal government site.
The site is secure. The https:// ensures that you are connecting to the official website and that any information you provide is encrypted and transmitted securely.
- Publications
- Account settings
- Browse Titles
NCBI Bookshelf. A service of the National Library of Medicine, National Institutes of Health.
Ackerman S. Discovering the Brain. Washington (DC): National Academies Press (US); 1992.

Discovering the Brain.
- Hardcopy Version at National Academies Press
8 Learning, Recalling, and Thinking
The brain regulates an array of functions necessary to survival: the action of our five senses, the continuous monitoring of the spatial surround, contraction and relaxation of the digestive muscles, the rhythms of breathing and a regular heartbeat. As the vital functions maintain their steady course without our conscious exertion, we are accustomed to consider the brain as preeminently the organ of thought . The brain houses our mind and our memories, and we rely on its information-processing capacities when we set out to learn something new.
But where in the brain can we locate memory or thought itself? Chapter 7 offered some clues about the ways scientific investigation—from the molecular level to studies of the alert, behaving animal—has begun to define in physical terms an abstract quality such as "attention." Similar techniques and approaches are being applied to other mental functions, too, even those as seemingly intangible as learning, remembering, or thinking about the outside world.
Learning and memory, which for many years were considered central problems in psychology, the social sciences, and philosophy, have recently assumed greater importance in the area of neurobiology, itself a confluence of several lines of investigation.
FIGURE 8.1.
Most available evidence suggests that the functions of memory are carried out by the hippocampus and other related structures in the temporal lobe. (The hippocampus and the amygdala, nearby, also form part of the limbic system, a pathway in the brain (more...)
Neuroscientific interest in learning and memory has recently increased for two reasons, according to psychiatrist Eric Kandel, a senior scientist in the Howard Hughes Medical Institute at Columbia University. One reason is the proposal of cellular mechanisms that account for a basic kind of learning and long-term memory. The model was first identified in the relatively simple nervous systems of the marine snail and the crayfish, but it appears to hold good in the hippocampus of vertebrates as well, where it also may be associated with the formation of long-term memories.
The second reason for a new interest in learning and memory is the evidence accumulating to suggest that mechanisms involved in the structural change in the nervous system that accompanies learning may strongly resemble certain important steps in the nervous system's development . In other words, the sorts of adjustments among synapses that account for learning may be the same as the "fine-tuning" that occurs while the maturing system is assuming its unique elaborated form. Thus, the biological changes that accompany learning may be seen—in a very schematic way—as an old process put to a new use, or as a specialized way in which the brain continues to "grow" after maturation.
- A Molecular Account Of Long-Term Memory
Eric Kandel is best known for his work on the physical basis of learning and memory in the marine snail Aplysia . This animal, simple as its nervous system is (most of its 20,000 neurons have been identified by number), nevertheless provides an excellent model for the study of learning and memory, through its "gill withdrawal" reflex. When Aplysia perceives something touching its skin, it quickly withdraws both the siphon (a respiratory organ) and the gill, much as a person withdraws a hand from a hot stove without thinking about it. Although this withdrawal is a reflex, it is not completely hard-wired but can be modified by various forms of learning. One such form is sensitization, in which the animal becomes aware of a threatening factor in the environment and to protect itself learns to augment its reflex. The augmented version of the withdrawal reflex can also be maintained in short-term or long-term memory, depending on whether researchers administer the noxious stimulus (the negative reinforcement) only once or twice, or many times within a short period. The two forms of memory can be distinguished not only by their duration—the difference between minutes and days—but also at a molecular level, because it is possible to treat the snail with a chemical compound that interferes with long-term memory but leaves short-term memory unimpaired.
A major set of elements in this reflex are sensory neurons in the siphon skin, which perceive the stimulus; motor neurons in the gill, which contract the muscle and cause the gill to withdraw; and "facilitating neurons," or interneurons, which act on the sensory neurons to enhance their effect. The role of these facilitating neurons has recently become clearer, thanks to observations made from cell cultures, at the simplest level possible: the neurons themselves. A single sensory neuron and a single motor neuron, when implanted in a glass dish with a suitable nourishing culture, form functional interconnections. When a facilitating neuron is added or the cells are exposed to serotonin (the transmitter released by the facilitating neuron), the connection between the sensory and the motor neuron becomes stronger. The connection can last in this enhanced form for more than a day, even up to several weeks, and apparently includes some process of genetic transcription, or expression of part of the nerve cell's DNA.
This genetic transcription produces two results that set long-term memory apart from short-term memory. One is a sort of extension of a short-term effect, in which the potassium channels in the sensory neuron membrane remain closed for a longer time, while the calcium channels remain open. The net effect is that the sensory neuron is more easily excited and releases more neurotransmitter, which in turn activates the motor neuron more strongly. Actually, this effect can be produced on a short-term basis by increased levels of the second-messenger compound cyclic AMP; but after transcription, it is no longer dependent on such a factor and persists even without it. The effect can be disrupted, however, by inhibitors of protein synthesis and RNA synthesis. This constraint establishes that the recording of long-term memories involves not simply a momentary release of neurotransmitters but actual gene expression, with the synthesis of new proteins in the nerve cells themselves.
The new protein products that are synthesized—for example, under the stimulus of a repeated threatening signal—do more than merely reduce the dependence of the sensory neurons on serotonin or cyclic AMP for their activation. As a second transcription event, they induce new growth in certain parts of the sensory neurons themselves. These neurons develop many more presynaptic terminals, the structures through which they release neurotransmitter to the motor neurons; in addition, the number and the surface area of active zones in each presynaptic terminal increase, as does the total number of vesicles, the storage containers for the neurotransmitter. Thus, gene expression appears to build long-term memory out of several effective components, which come together in a formidable array: increased excitability of the sensory neurons, with the protein kinase continuing to work on its own to keep calcium channels open, allowing calcium ions in and more neurotransmitter out; more synapses for conveying signals between sensory and motor neurons; greater numbers of active zones in the synapses; and greater quantities of neurotransmitter contained in the active zones, ready for release. No wonder that memories built of such stuff tend to last awhile.
For closer study, the Kandel laboratory has replicated in cell culture the same conditions that in the living animal lead to protein synthesis and neuronal growth: a motor neuron, a sensory neuron (injected with a fluorescent dye to make imaging possible afterward), and exposure to serotonin repeated four or five times. The results are clear: within several hours, the main axon of the sensory neuron shows an increase in the number of synapses. Exposing the neurons to the second messenger cyclic AMP produces a similar result. But regardless of whether the facilitating compound is the neurotransmitter or the second messenger, neuronal growth occurs only if a target—a motor neuron—is also present.
The necessary presence of a target was the first similarity that Kandel and his collaborators noticed between the processes of structural change that accompany long-term memory, or learning, and those of development. The observation fit in well, too, with an earlier finding: the fine axonal branches of a sensory neuron in isolation adhere together in fat bundles, but on first contact with a motor neuron the branches tend to separate, each potentially to form its own synapse with the motor neuron. Here, at a mechanical level, is the explanation for a disassembly process that is required prior to the marked increase in synapses that takes place in the presence of serotonin. But in long-term memory, as in development, the presence of the target is necessary—a feature that makes for plasticity, or the all-important ability to change in response to the environment.
To study this learning-related plasticity at the molecular level, Kandel's research group is looking at the proteins that change in level when exposed to serotonin or cyclic AMP (or, in the living animal, to a noxious stimulus). Of the 15 proteins that change, 10 show an increase and 5 show a decrease. The reactions are transient: the levels go up, or down, and back again quite quickly.
Most interesting, in the investigators' view, are the proteins whose response is to decrease in level. Is there a way in which producing less of something can figure in a growth process? At a molecular level, the answer can be yes, if the something is an inhibitory factor of some kind. Such an answer may apply in this case, because four of these proteins that have been identified by genetic sequencing turn out to be none other than cell-adhesion molecules of the immunoglobulin type, first discovered by the research team of Gerald Edelman at Rockefeller University.
During development, the proteins apparently play a fundamental role; at least one of them is present at the very first stages, when the fertilized egg begins to divide. In the adult, however, these four proteins appear only in the nervous system, in both sensory and motor neurons. An interesting effect of these cell-adhesion proteins can be demonstrated on an isolated sensory neuron: if an antibody is added that blocks the cell-adhesion effect, the axonal filaments of the neuron start to come apart from their thick bundle and to separate out. The effect is similar to what happens when a sensory neuron is exposed to serotonin in the presence of a target, a motor neuron. This suggests that cell-adhesion molecules can indeed act as an inhibiting factor in particular circumstances. What they inhibit, apparently, is the growth and proliferation of signal-transmitting elements on the axons of sensory neurons.
By this reasoning, the effect of the cell-adhesion molecules would have to be held in abeyance at some point, to allow the sensory neurons to strengthen and increase their synaptic connections with the motor neurons. Perhaps there is even an innate tendency for some neurons, when they are near other target neurons, always to have their axons branching and proliferating, always to be seeking to form more synapses. (Indeed, during development, as discussed in Chapter 6 , the brain actually forms a great many more synapses than can ever be functional during the animal's lifetime.) The inhibitory action of the cell-adhesion molecules may thus be a crucial factor that keeps neuronal growth somewhat under control, and the temporary inhibition of cell-adhesion molecules in favor of long-term memory may be a single, notable exception to this form of containment. Of course, these results come from painstakingly close study of very simple nervous systems. The degree to which such findings can be extrapolated to the brains of primates, for example, which are many times more complex and which follow different patterns of development, is a matter of lively discussion among researchers in various specialized areas of neuroscience.
One striking aspect of such a system is the ingeniously high level of what, in a person, might be called thriftiness—the degree to which the same materials or biological processes are used and reused, but in novel contexts and to different ends. The protein kinase described earlier, which is dependent on cyclic AMP, appears in many other systems of the body and has various effects; but only in the nervous system, in relation to learning, does it play a role in long-term activation. Likewise, cell-adhesion molecules—better known to researchers for their general role in development—play a rather specialized part in the adult nervous system.
Just as intriguing, from a different perspective, is the evidence for significant common ground between biological mechanisms of learning and the early development of the organism: not only the common use of cell-adhesion proteins (although in different ways) but also the fact that growth in both contexts requires a target. Even the finding that a neurotransmitter such as serotonin is not restricted to moment-by-moment signaling but can actually be a factor that initiates neuronal growth in the case of long-term memory adds to an impression of the two contexts conjoining, with neurotransmitters sometimes acting as growth factors.
- The World In The Front Of The Brain
Short-term and long-term memory are not the only forms in which the brain stores information. All the time that the five senses are operating, the brain is assembling and sorting perceptions of the outside world, directing some to conscious attention and collecting others into a set of perpetually updated mental representations. Although we may seldom be aware of the full extent of these mental representations, or examine them directly, nevertheless, they hold great importance for our thought processes and our ability to carry out the simplest planned action or predictive step, even something as elementary as following a fast-moving target with our eyes. These mental representations are the data on which we base cognition—our thoughts, ideas, and abstract mental processes.
Animals, too, form complex mental representations of the world, which are shaped by their own brain structure and ecological requirements. For instance, information gathered through the sense of smell undoubtedly plays a much larger role in the mental representations of a dog than in those of a bird, which relies much more on its excellent vision (both in detail and in color) to help it recognize its kin, observe the territories of its rivals, and seek out food and mates. With such differences taken into account, the study of mental representation in animals can help scientists explain similar processes in humans, particularly if the neurobiology of the animal is also under study or is well known from earlier research.
Mental representation in the monkey, in the form of short-term or working memory, has actually been studied for more than 50 years. The earliest experiments were carried out as delayed-response tests: the monkey was shown a morsel of food being placed in one of two food wells and after a short delay had to open the correct one to claim the food as a reward. The reason for the delay was to force the monkey to rely on an internal mental representation rather than on immediate stimulation—that is, what it saw taking place at that moment. In the rhesus monkey, the area of the brain known to be important for this task is the prefrontal cortex; and in humans, too, homologous areas in the frontal region of the cortex, just behind the forehead, are sites of activity for tasks that test working memory.
Present-day research of this kind with monkeys uses a computer monitor. In such experiments, the animal directs its gaze to the center of the screen. While it keeps its attention fixed on the central spot, a visual target (a light) flashes briefly (for half a second) somewhere else on the screen. The monkey's task (which requires some months of training) is to keep its eyes fixed on the central spot as long as it is lit, and then, when the central spot has been switched off, to move its eyes to the place where the visual target had flashed some seconds before. Clearly, the test calls for working memory: the chances of turning one's eyes to the correct site by a lucky guess are slight, and since the visual target can appear anywhere at all on the screen, in any sequence—not simply location A alternating with location B—there is no possible way to ''prepare" the correct response beforehand. A monkey that is practiced in this task can perform with a high degree of accuracy; but when a portion of its principal sulcus is removed by surgery, an animal that was previously proficient performs with no more than 50 percent accuracy.
Given this sharp drop in performance, what is the nature of the deficit in the monkey's brain after surgery? Patricia Goldman-Rakic, who directs such investigations at Yale University Medical School, explains that it can be considered a "hole" in the memory—not in vision or in the ability to move the eyes. These faculties show up unimpaired in tests in which the visual target is left on (so that the monkey simply moves its eyes to the target at the appropriate time). Only the ability to guide the response by a mental image (memory) is missing.
Another complementary way of investigating the same topic is to record electrical activity from the brain during a working memory task. The ideal record in terms of clarity and precision is one obtained from a single neuron, by means of extremely fine microelectrodes. Recordings of this kind have become possible only in the past decade or so; those from Goodman-Rack's laboratory show several very interesting things. First, the neuron under study, in the prefrontal cortex, holds to a steady level of activity when the target light appears. But it increases its activity sharply once the target light is switched off and shows sustained activity during the delay, the interval over which a memory of the target must be maintained. Finally, the neuron's activity rather abruptly returns to a baseline level when the monkey begins its response—that is, when it moves its eyes to the site where the target had been. The neuron thus shows a high level of activity only during the time required to keep the correct spot "in mind" until the moment arrives to respond actively.
A second point of interest from these recordings is that the neurons of this region in the prefrontal cortex each tend to remember one precise location on the screen—and no others. For example, one neuron would respond accurately for targets at a 270-degree rotation from the center but would remain unresponsive to all other locations; another neuron would respond only to targets at a 90-degree rotation. In an analogy with the visual system, the neurons form a "memory field" in much the same way that nerve cells of the occipital lobe form a visual field. The memory field even shows the same cross-brain pattern that is traced by many signals: neurons oriented to the memory of stimuli that appeared in the right visual field predominate in the left hemisphere, and those oriented to the memory of stimuli presented in the left visual field predominate in the right hemisphere.
In Goodman-Rack's words, memory is an added-on feature of the representation system for visual space. Bearing out this interpretation are recordings from trials during which monkeys that were usually accurate made a mistake in their response, moving their eyes to the wrong place. The electrical data show that the particular neurons for that location were not highly active during the delay period, and so they failed to sustain the mental representation.
According to a current view, these neurons are organized in modules rather like the ocular dominance columns of the visual system. Several lines of research have established that the principal sulcus receives a great deal of its information about the outside world from the parietal cortex, which specializes in visual spatial information (as discussed in Chapter 7 ). The nerve tracts that project from the parietal lobe do in fact form a pattern of columns in the prefrontal cortex that alternates with columns for incoming signals from other regions. As in the visual system, each column is about half a millimeter wide.
These mental representations in the prefrontal cortex are too limited to be directly responsible for an animal's complex behavior. Goldman-Rakic and her colleagues believe that this representational knowledge does guide behavior in collaboration with other areas—particularly the parietal cortex—and that the larger network very probably represents the neural circuitry underlying spatial cognition in monkeys. Different parts of the network, and the connections among them, must be analyzed separately before the ensemble can be well understood as a network. A broad assortment of psychological studies have shown that when people are asked to perform any cognitive task, the prefrontal cortex invariably is activated; what remains to be discerned is which particular subdivisions of the area (visual or auditory or other) are involved. Increasingly specific testing, anatomical examination, and medical imaging of animals and human subjects are the tools that can provide this kind of information.
Meanwhile, noninvasive medical imaging of humans offers opportunities for the direct simultaneous study of physiology and mental functioning. In addition to NMR and PET scans, electroencephalographic studies can be quite useful, recording electrical activity at the scalp with great temporal precision. Recent EEG studies have shown that when a subject performs cognitive or judgment tasks that require keeping something in mind over a short period, a number of areas in the prefrontal cortex are active. When, on occasion, the subject makes an error, it appears that the network as a whole was not engaged.
- Neurotransmitters And The Information System
In addition to the information-processing circuits arranged in neuronal modules and in columns of incoming nerve tracts, the brain is replete with other systems of input. In the prefrontal cortex, for example, nerve fibers containing the neurotransmitter dopamine are found in especially high concentration, and researchers have wondered for some time what role dopamine might play in prefrontal circuits of information. The evidence gathered on this point over the past few years has begun to make clear the enormous extent to which dopamine shapes not only our physical functioning in the world but also our ability to process new information, to associate ideas effectively, and even to maintain a sense of well-being in balance with realistic perceptions.
In the human prefrontal cortex, the nerve fibers containing dopamine are not scattered evenly throughout the six cerebral cell layers but are concentrated in the outermost layers and the deep layers—that is, in layers 1, 5, and 6—and are less densely distributed in the middle layers. The cell bodies of these neurons are located relatively far away in the ventral tegmental area, a portion of the brainstem; they preferentially project their fibers to the frontal and prefrontal cortex. In addition, researchers have identified at least two distinct kinds of receptor sites for dopamine, and each has its own pattern in the layers of the cortex. The preponderance of the D-1 receptor fairly matches that of the dopamine-containing fibers: very high in the outermost layers and also considerable in the deep layers. The D-2 receptor, by contrast, shows a lower concentration throughout, with just a mild peak in layer 5.
In a test to see whether interference with the D-1 receptors would have any effect on cognitive function, Goldman-Rakic's research team injected a compound that blocks the D-1 receptor sites in the prefrontal cortex of monkeys trained in the delayed-response test described earlier. About 20 minutes after the injection, the animals showed an impairment of working memory, moving their eyes to the wrong location when the trial included a delay; but they responded correctly in a "sensory-guided" version of the task, in which the target light was left on as a guide. The D-1 receptors thus appear to be implicated in the efficiency of working memory.
A chemical compound developed for use in research that selectively stains neurons in the cerebral cortex bearing D-1 receptor sites has provided the Yale research team with an interesting lead. These neurons have been identified as pyramidal cells, the large principal cells that are the main element of cerebral cortex layer 6. The axons of these cells carry signals to another region—in this case, the thalamus (which plays an important role in the control of movement and forms part of the limbic system).
It appears from electron-microscopy studies that the dopamine receptors on these cells may modulate excitatory synapses, possibly from other pyramidal cells in the same or another region. Therefore, since dopamine acts directly on the output neurons of the prefrontal cortex—which are involved in processing, sorting, and assembling information about the outside world—the dopamine circuits can be considered a physical pathway by which this neurotransmitter can influence cognitive function. With each neuron bearing millions of spines on which dopamine synapses may act, a mechanism of this kind can have a pervasive effect, and even a slight deficiency or excess of dopamine could powerfully alter the ability of many neurons to integrate information from other regions of the brain. Goldman-Rakic and her colleagues are looking closely at the identified dopamine synapses to understand more precisely the mechanism by which dopamine may affect cognition.
The prefrontal cortex, with its importance for cognition, shows a form of dysfunction when tested in patients suffering from schizophrenia. (An often disabling mental illness, schizophrenia interferes with the capacity for logical thought and greatly disturbs the emotions and social behavior; see Chapter 4 for a discussion of current theories about the importance of dopamine levels in schizophrenia.) In experiments calling for cognitive tasks, which normally require the participation of the prefrontal cortex, schizophrenic patients show significantly lower rates of activity in this region of the brain. This does not mean that a disorder as complex and varied in form as schizophrenia can be explained as a simple failure of one part of the brain—particularly since the prefrontal cortex is known to be so richly interconnected with many other regions. But the findings that indicate a less active prefrontal cortex, which have been replicated in numerous studies, fit in well with other evidence suggesting that some dysfunction in a network of areas, including the prefrontal cortex, is implicated in schizophrenia.
Studies are under way to probe the state of working memory in schizophrenic patients as a way of learning more about the normal and impaired functioning of the prefrontal cortex. Meanwhile, rhesus monkeys treated in such a way as to mimic some of the deficits characteristic of schizophrenia are also being tested for working memory, thereby allowing more direct study of the neurobiology involved. One of the behavioral deficits that has been experimentally produced in monkeys is the inability to track a fast-moving target with the eyes. The deficit is not based in the visual or motor system; this much is clear, because the monkeys remain able to track targets moving more slowly. Instead, the problem seems to be cognitive, an inability to predict where the target will be in the next fraction of a second. This predictive aspect of eye movements, which falters in schizophrenic patients and in the experimentally treated animals, may well draw on the type of mental representations that the prefrontal cortex is largely occupied in assembling. The research being conducted in animals and humans is mutually helpful, offering the prospect over the next decade of significant advances in a neuroscientific account of the workings of the prefrontal cortex—including a cellular explanation of this area's memory functions. A view shared currently by Goldman-Rakic and many colleagues is that the main function of this greatly enlarged part of the brain, so recently evolved in the primate line, is to guide behavior by means of mental representations of stimuli, rather than by the stimuli themselves. Over the course of primate evolution, the advantages of this mode of mental functioning would have been considerable, greatly expanding the animal's options for varied and complex behavior.
- What Kind Of Computer Is This?
The types of mental representation discussed above, such as the continuous monitoring of the spatial surround by the parietal lobes, illustrate a vital point that is often overlooked when comparisons are made between the human brain and the computer. The fact is that the human brain—or the brain of many other animals—is solving quite difficult computational problems at every moment, just in seeing, recognizing a voice, or moving in a coordinated fashion on four limbs, or two limbs, or two wings. Most of these problems are so complex that they have yet to be formulated in explicit terms by computer scientists, which is why machines that can perceive and move and communicate as animals do—and perform all these functions at once—are still largely the stuff of science fiction.
If computers are not really brains, what does it mean to call the brain a kind of computer? Terrence J. Sejnowski, whose work at the Salk Institute for Biological Studies in San Diego focuses on computer models of cognition and brain structure, answers this question by pointing to a simple device designed to do one thing optimally, and one thing only: play tic-tac-toe. This "computer," built from electronic Tinkertoys at MIT's Artificial Intelligence Laboratory, is programmed with every possible position in the game. (These have been reduced, through mathematical operations that apply the principle of symmetry, to a subset of about 48.) The positions, each with its one optimal response, are encoded as the computer's memory. When presented with a particular position, the computer matches it to one in its subset and produces the correct response. By contrast, a digital computer would meet the challenge with a set of programmed instructions that it would run through recursively at each move to arrive at the optimal response.
The MIT device does not carry out a string of calculations or algorithms, the kind of task we generally think of a computer performing; instead, what it offers is essentially a "look-up table," with the correct answer precomputed and readily available. To obtain swift access to that answer, however, one must present a problem that exactly matches one of the problems originally encoded in the computer's memory. Beyond that pre-encoded set, the computer cannot provide any correct answer—or even a partial answer—unlike a digital computer, which can be reprogrammed for new problems because of its more general mode of operation. Still, within the realm of its pre-encoded problems and responses, the "look-up table" is extremely fast and effective.
This kind of device, however, requires a great deal of memory, since every significant aspect of each pre-encoded problem must be specified if the match is to be accurate. For the game of tic-tac-toe this is manageable; for chess, with its 10 40 possible game positions, or for real-life contexts in which the rules are less clear, it is impossible, at least at present. As a practical device, the look-up table is strictly limited. However, the principle of precomputing certain responses and being able to retrieve them with minimal additional effort appears to Sejnowski and others in his field as a likely clue to some of the workings of the brain. True, the abundant memory required by a look-up table was extremely expensive in the first computers and still poses a practical challenge today. But if the amount of memory were tremendously expanded, it would be possible to store many more solutions—in other words, to address many more and different kinds of problems.
It is hardly a revelation at this point that the human brain exhibits just such a tremendous capacity to store information. With somewhere between a hundred billion and a trillion neurons, the human brain already looks fairly impressive—but what really expands its storage capacity far beyond anything we can yet envision on an engineer's drawing board is the brain's proliferation of synapses. Each neuron contains several thousand points at which signals can be transmitted. Even if the brain were to store information at the low average rate of one bit per synapse (in terms comparable to a digital code, the synapse would be either active or inactive), the structure as a whole could still build up vast stores of memory, on the order of 10 14 bits. Meanwhile, today's most advanced supercomputers command a memory of about 10 9 bits. The human brain, to use Sejnowski's phrase, is memory-rich by comparison.
Of course, organization is crucial to managing such a vast resource, and the brain exhibits this feature at several levels, as discussed throughout this book. Research conducted on the simpler nervous system of invertebrates, as well as on nonhuman primates, other vertebrates, and humans, has indicated how learning brings about structural changes in nerve cells and how the neurons in turn form regions, which take part in networks. The networks are organized into distributed systems, which collaborate with other systems, both sensory and associative, to produce the total working effect.
Memory itself is organized so as to take advantage of these many levels of information: it appears to be arranged along associative paths, by the principle of contiguity. That is, the brain associates bits of information in such a way that we can recall items either on their own or by being "reminded" of them by a cue. The name of an acquaintance may come to mind when needed, or we may search for it under one heading or another: the name sounded like that of another friend, or the person looked like a former co-worker, or the meeting took place at the lunch following a difficult business negotiation. Considering the brain in purely physical terms, researchers have suggested that another form of contiguity may apply as well, that is, the simple proximity that builds up into maps. It may be that neurons close enough to one another to be activated together keep some trace of that contiguity as part of their bit of information.
Just what the memory-forming mechanisms might be, at a physiological level, has long puzzled psychologists as well as neurobiologists. Evidence of several kinds is gathering, however, in support of a model first suggested in 1949 by Donald Hebb, that a memory forms as a result of at least two kinds of activity taking place at a synapse simultaneously. The activities would have to include both the preand postsynaptic elements, the neuron transmitting the signal and the one receiving it. Hebb reasoned that the strength of the signal received in the postsynaptic cell would depend on the interaction of many details—the amount of transmitter released, the presence or absence of neuromodulators that affect the postsynaptic cell's excitability, the number of receptor sites on the receiving cell, and other such variables. Whatever the specifics, the underlying principle would be that information is stored as a result of two or more biochemical factors coming together in time, at the same instant, and in space, at the same synapse.
Physical evidence that indirectly supports this model has come recently from Eric Kandel's work with Aplysia . Hebb postulated two active elements (the pre- and postsynaptic terminals), but the nervous system in the marine snail appears to include a third element, the facilitating neuron that enhances the excitability of the sensory neuron. The Hebbian principle still applies, however, to the extent that the variables have to meet in time and space at a synapse.
In mammals, an example that conforms even better to the Hebbian model is found in part of the hippocampus of rats. The particular area, designated CA-3, contains about half a million neurons with recurrent connections—in other words, many of their axons lead back into the same population of neurons. Some axons also lead into the adjacent area CA-1. At the synapses in this area, both among CA-3 cells and between CA-3 and CA-1 cells, the neurotransmitter glutamate is released. It binds to two types of receptors: at one type of receptor site the glutamate slightly lowers the excitability threshold of the neuron, but at the other the binding of glutamate does not in itself affect the cell. Another simultaneous event is required: depolarization of the receiving cell, perhaps by other synapses. When this occurs together with the binding of glutamate, the cell membrane becomes momentarily permeable to ions—particularly calcium ions, which are important for bringing about persistent changes in the structure of the cell.
This receptor system illustrates the principle of contiguity outlined by Hebb: the binding of glutamate to a particular kind of receptor site and the depolarization of the postsynaptic cell must occur simultaneously, or at least within the same 20 to 50 thousandths of a second, for calcium ions to enter the cell and induce structural changes.
- Assembling A Brain In The Laboratory
Hebbian synapses have also been demonstrated in another kind of laboratory, where computer scientists and engineers have built them into a computer chip. The device is a simple one, with only 16 synapses, but it performs Hebbian learning quite efficiently, at the rate of a million times per second. Newer chips have already been developed to represent more realistic neurons, with many thousands of synapses; and technology to represent the connections between such neurons will make the assembly of something more nearly resembling a working brain a little easier to envision. Such a device will have to combine analog signals, like those propagated within neurons, and digital signals, the off or on impulses transmitted from one neuron to another. It will not be simply a larger, or even an unbelievably faster, version of today's familiar computer.
An artificial brain of this kind could be invaluable for further research along two main lines. For one, it could be set to work on some of the more difficult problems in an emerging field that might be called "artificial perception": problems of computer vision and of speech recognition that can be delineated by current devices but that cannot be resolved by them in a practical way. For a second main line of research, this kind of artificial brain can offer an advanced testing ground for neuroscientists' ideas about how the brain functions. Theoretical models of memory, in particular, cannot be tested adequately on a digital computer simulation of a few hundred model neurons, because the living brain works on such an enormously larger scale. But a computerized circuit of several million model neurons, with information circulating in real time, could yield a whole new order of information about such circuits in the living animal.
The field of artificial perception already boasts chips developed at the California Institute of Technology that are capable of much of the sensory processing performed just outside the brain by the retina, for example, and by the cochlea, the spiral passage of the inner ear whose hair cells respond to vibrations by sending impulses to the auditory nerve. Now in development as well are chips to simulate some of the functions of the visual cortex; others, with some of the memory-storing capacity of the hippocampus, are being scaled up, closer to the dimensions of a living system.
But more time and knowledge are needed to produce a device that can successfully mimic the information processing of the five senses and of short-term and long-term memory, and that can, moreover, integrate these systems into a unit that functions as a whole with respect to the outside world. This is not to say that progress has not occurred: early computers of the 1950s carried out only a few thousand instructions per second (a speed matched by today's pocket calculators), whereas the fastest of the supercomputers in use today can perform billions of operations per second. Still, this rate of processing, at 10 9 or so operations per second, is far from that of the human brain, in which an estimated 10 14 synapses are each active about 10 times per second—giving a total of 10 15 operations per second.
An interesting constraint that confronts computer designers who work with the current top speeds is the simple, unchanging limitation posed by the speed of light. Signals simply cannot be transmitted faster than about 1 foot per billionth of a second (10 -9 , the speed of light); to achieve the effect of speeds higher than this, the computer must be reduced in size to less than a cubic foot. This reduction is made possible by duplicating the central processor many times, even thousands of times, within the same computer, so that signals have less distance to travel. Even so, extrapolating from the recent rate of increase and from today's highest known speeds of computer processing, Terrence Sejnowski estimates that an artificial device approximating the human brain cannot be expected before at least the year 2015.
This prediction should not be considered discouraging—far from it. For such a project to be within sight at all is the clearest possible sign of the progress of neuroscience, gaining impetus as it does from an increasing number of fields that are related in some way to its investigations. Now not only the biological sciences, medicine, biochemistry, pharmacology, and psychology have an interest in improving our understanding of the brain's functioning; the computer sciences, physics, and mathematics also contribute to such models and stand to gain much from their continued exploration and testing. And along the way toward the assembly of a fully functioning artificial brain, it should become increasingly possible to construct devices that satisfactorily replicate some of the principles at work in the human brain. Although the devices probably would not resemble a brain in their material form any more than an airplane resembles a bird, they will be successful if they can show some of the brain's operating principles adapted to their own form, just as an airplane carries out, in mechanical translation, some of the aerodynamic principles of natural flight.
- The Benefits Of An Artificial Brain
Of course, the brain cannot ever be completely characterized in terms of a computer because in addition to all its computing faculties it possesses the properties of a biological organ in a living system. But, points out Gerald Edelman of the Neurosciences Institute at Rockefeller University, computers can indeed do something that, until recently, only a brain could do: they can carry out logical functions. Today, a computer can address any challenge or problem that can be described in a logical formula. This still leaves unexplored vast areas of human experience, such as perception; but as described earlier in this chapter, computer and mathematical modeling on one side, and more detailed neurobiological examination on the other side, are making inroads in this area too.
Edelman and his colleagues have used an approach they call synthetic neural modeling to build an automaton that is able to explore its environment by simulated vision and touch; moreover, it can categorize objects on the basis of its perceptions, and its responses draw on previous experiences with similar objects. Darwin III (the third generation of its kind) is a robot whose nervous system is built of about 50,000 cells of different types. The signals transmitted at its approximately 640,000 synaptic junctions enable Darwin III to control the functioning of its one eye and its multijointed arm. In analogy with the way living brains enter the world, Darwin III has no specific information built into its systems about the objects it may encounter in its environment. The nervous system is pre-encoded only to the extent that the devices for perception are made to detect certain features, such as light or movement or rough texture.
An important principle of Darwin III's nervous system is that the strength of the synaptic connections can increase selectively with greater activity when that activity leads to an adaptive end. What is ''adaptive" for Darwin III is defined by arbitrary values built into its programming. For example, the built-in principle that light is "better" than no light serves to direct and refine the system's eye movements toward a target. Just as in living neurons, the enhanced connection provides a stronger response the next time that particular neural pathway is active.
This selective strengthening of connections is reminiscent of the competition among synapses in the developing brain (as discussed in Chapter 6 ). Together with the ability to categorize, it means that the system can produce behaviors that we commonly call "recognition," for instance, or "association." At present, Darwin III can turn its head to track a moving object with its eye; it can extend its arm to trace the contours of an object; and, alternatively, if the stimulus is noxious, it can swat the object away. In all these responses the system shows increasing accuracy with practice, as the relevant synapses are strengthened. Eventually, such a system should be able to teach itself to apply both visual and motor abilities to a complex task—for instance, distinguishing a particular object or kind of object, and picking it out with the arm from among many others.
Although Darwin III cannot represent the nervous system of living animals in a highly detailed way, its synapses and circuits provide a much-needed testing ground for ideas about what takes place inside the real thing that makes those 3 pounds of semisoft tissue the most complex information-processing system ever known. Perhaps computers can never be brains in the full sense of serving as the nervous center of a biological system, but they can be designed with increasing success to carry out some of the functions that are routinely managed by a living brain. Gerald Edelman, like Terrence Sejnowski, believes that the prospects for building more complex "perception machines" are good—and the benefits in both intellectual and economic terms will be enormous. Most important of all would be the expanded opportunities for an understanding of higher brain functions—those that make us human—to be gained by using the computer not so much as a model of the brain, but as a tool for exploring it.
- Acknowledgments
Chapter 8 is based on presentations by Gerald Edelman, Patricia Goldman-Rakic, Eric Kandel, and Terrence Sejnowski.
- Cite this Page Ackerman S. Discovering the Brain. Washington (DC): National Academies Press (US); 1992. 8, Learning, Recalling, and Thinking.
- PDF version of this title (3.2M)
In this Page
Recent activity.
- Learning, Recalling, and Thinking - Discovering the Brain Learning, Recalling, and Thinking - Discovering the Brain
Your browsing activity is empty.
Activity recording is turned off.
Turn recording back on
Connect with NLM
National Library of Medicine 8600 Rockville Pike Bethesda, MD 20894
Web Policies FOIA HHS Vulnerability Disclosure
Help Accessibility Careers
- Type 2 Diabetes
- Heart Disease
- Digestive Health
- Multiple Sclerosis
- Diet & Nutrition
- Supplements
- Health Insurance
- Public Health
- Patient Rights
- Caregivers & Loved Ones
- End of Life Concerns
- Health News
- Thyroid Test Analyzer
- Doctor Discussion Guides
- Hemoglobin A1c Test Analyzer
- Lipid Test Analyzer
- Complete Blood Count (CBC) Analyzer
- What to Buy
- Editorial Process
- Meet Our Medical Expert Board
The Anatomy of the Prefrontal Cortex
Associated conditions, frequently asked questions.
The prefrontal cortex is an important part of your brain. It is at the front of the frontal lobe, which is immediately behind the forehead. It affects your behavior, personality, and ability to plan. This article will explain more about the anatomy, location, and function of the prefrontal cortex.
Eric Raptosh Photography / Getty Images
The prefrontal cortex (PFC) is connected to many other parts of the brain and is able to send and receive information. The prefrontal cortex is divided into these two parts:
- Medial PFC (mPFC) : It is involved in self-reflection, memory, and emotional processing.
- Lateral PFC (lPFC) : It is involved in sensory processing, motor control, and performance monitoring.
The prefrontal cortex is involved in many brain functions. One of the most important is executive function , or the ability to self-regulate and plan ahead. Examples of executive function include:
- Controlling your behavior and impulses
- Delaying instant gratification
- Regulating your emotions
- Making decisions
- Solving problems
- Making long-term goals
- Balancing short-term rewards with future goals
- Changing your behavior when situations change
- Seeing and predicting the consequences of your behavior
- Being able to consider many streams of information
- Being able to focus your attention
The prefrontal cortex also affects your personality. A historical example of what happens when a person’s prefrontal cortex is damaged occurred in the mid-1800s. When railroad worker Phineas Gage’s prefrontal cortex was damaged by a metal rod going through his skull, he survived, but his personality changed. He became impulsive and lost the ability to plan.
Damage to the prefrontal cortex can happen from:
- Brain trauma : Accidents, falls, sports injuries, and physical altercations can cause a traumatic brain injury.
- Cancer : Cancer originating in the brain (primary tumors) or spreading to the brain from other original sites ( metastatic brain tumors ) can cause damage.
- Tumors : In addition to cancerous tumors, benign (noncancerous) brain tumors can harm the prefrontal cortex.
- Stroke : A blocked blood vessel or bleed in the brain can damage the prefrontal cortex.
When the prefrontal cortex is damaged, it may cause the following conditions:
- Changes in personality and behavior
- Problems with social behavior and an increase in antisocial behavior
- Higher chance of committing violence or stealing
- Problems regulating emotions and impulses
- Attention deficit hyperactivity disorder (ADHD) : A neurodevelopmental condition that affects attention, hyperactivity, and impulsiveness
- Post-traumatic stress disorder (PTSD) : A mental health disorder that affects people after traumatic events
- Schizophrenia : A mental health condition that affects a person’s behavior, thoughts, and feelings
- Bipolar disorder : A condition that causes extreme mood swings
If you have damage to the prefrontal cortex or another condition that is affecting it, your healthcare provider may start with a physical exam and a mental status exam. These tests will help them evaluate your thinking and rule out other conditions.
To check your brain, a healthcare provider may order the following tests:
- Magnetic resonance imaging (MRI) : Detailed images taken using magnetic fields
- Computed tomography (CT) scan : A detailed computerized X-ray scan
- Positron-emission tomography (PET scan) : Imaging that uses a radioactive tracer to look for cells that are active
The prefrontal cortex is found in front of the frontal lobe of the brain. It affects your behavior, personality, and executive function. When the prefrontal cortex is damaged, it can cause changes to how you think and behave.
A Word From Verywell
It is important to remember that you may not always notice changes in your behavior or thinking. Your friends and loved ones are more likely to point out that something is wrong.
Even if you think everything is fine, it is worth having a conversation with your healthcare provider and checking on your brain health. It is better to catch problems earlier and get treatment.
Yes, the prefrontal cortex grows as a person matures from childhood to early adulthood. It is one of the last parts of the brain to develop completely.
In general, the prefrontal cortex is considered fully developed by the age of 25. This is why car insurance companies charge higher rates until a person turns 25 because they are high-risk drivers. They explain that the prefrontal cortex is involved in risk-taking and decision-making, which are both important for driving.
A person may lose executive function if the prefrontal cortex is damaged. They may still be able to do some tasks and even work, but they will have trouble planning and controlling their behavior.
Yes, it is possible for a person to survive without a prefrontal cortex. However, not having this portion of the brain will have an enormous impact on their ability to reason, plan ahead, control behavior, and solve problems. A person’s ability to have social relationships would also be affected severely.
Grossmann T. The role of medial prefrontal cortex in early social cognition . Front Hum Neurosci . 2013;7:340. doi:10.3389/fnhum.2013.00340
Arain M, Haque M, Johal L, et al. Maturation of the adolescent brain . Neuropsychiatr Dis Treat . 2013;9:449-461. doi:10.2147/NDT.S39776
Teles RV. Phineas Gage's great legacy . Dement Neuropsychol . 2020;14(4):419-421. doi:10.1590/1980-57642020dn14-040013
Barrash J, Bruss J, Anderson SW, et al. Lesions in different prefrontal sectors are associated with different types of acquired personality disturbances . Cortex. 2022;147:169-184. doi:10.1016/j.cortex.2021.12.004
By Lana Bandoim Bandoim has nearly 20 years of experience writing for a variety of outlets including health sites, scientific publishers, and academic medical centers.
Connection denied by Geolocation Setting.
Reason: Blocked country: Russia
The connection was denied because this country is blocked in the Geolocation settings.
Please contact your administrator for assistance.
- Brain Development
- Childhood & Adolescence
- Diet & Lifestyle
- Emotions, Stress & Anxiety
- Learning & Memory
- Thinking & Awareness
- Alzheimer's & Dementia
- Childhood Disorders
- Immune System Disorders
- Mental Health
- Neurodegenerative Disorders
- Infectious Disease
- Neurological Disorders A-Z
- Body Systems
- Cells & Circuits
- Genes & Molecules
- The Arts & the Brain
- Law, Economics & Ethics
- Neuroscience in the News
- Supporting Research
- Tech & the Brain
- Animals in Research
- BRAIN Initiative
- Meet the Researcher
- Neuro-technologies
- Tools & Techniques
Core Concepts
- For Educators
- Ask an Expert
- The Brain Facts Book

Important Brain Regions for Decision-Making
- Reviewed 8 May 2023
- Author Marissa Fessenden
- Source BrainFacts/SfN
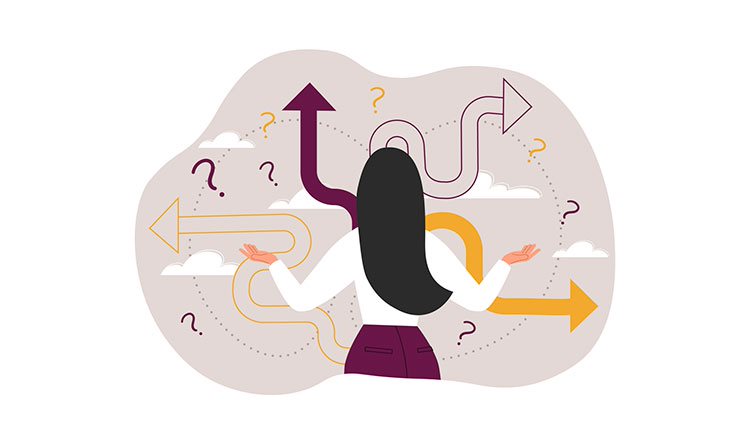
You make many different types of decisions every day. Some of these rely primarily on logical reasoning — for example, when you compare the timetables for the bus and subway to determine the quickest way to get to a friend’s house. Other decisions have emotional consequences at stake, like when the person you’re trying to impress offers you a cigarette — your desire to be accepted might outweigh your rational consideration of smoking’s harms.
Decision-making requires a person to weigh values, understand rules, make plans, and form predictions about the outcomes of their choices. Both logical reasoning and emotional (affective) decision-making involve the brain’s prefrontal cortex (PFC). In particular, activity in the lateral PFC is especially important in overriding emotional responses during decision-making. The area’s strong connections with brain regions related to motivation and emotion, such as the amygdala and nucleus accumbens , seem to exert a sort of top-down control over emotional and impulsive responses. For example, brain imaging studies have found the lateral PFC is more active in people declining a small monetary reward given immediately in favor of receiving a larger reward in the future. This is one of the last areas of the brain to mature — usually in a person’s late 20s — which explains why teens sometimes may have trouble regulating emotions or controlling impulses.
The orbitofrontal cortex , a region of the PFC located just behind the eyes, also appears to be important in affective decision-making, especially in situations involving reward and punishment. The area has been implicated in addiction as well as social behavior.
Adapted from the 8th edition of Brain Facts by Marissa Fessenden.
About the Author
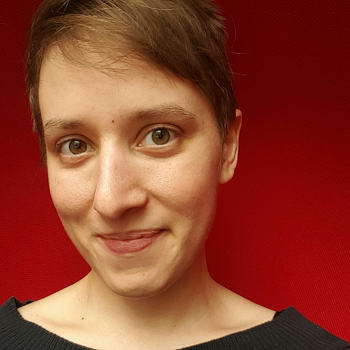
Marissa Fessenden
Marissa Fessenden is a freelance science journalist and illustrator. They gravitate toward stories about genes, wildlife and places large and small, as well as times when art gets science-y or science gets artsy.
CONTENT PROVIDED BY
BrainFacts/SfN
What to Read Next
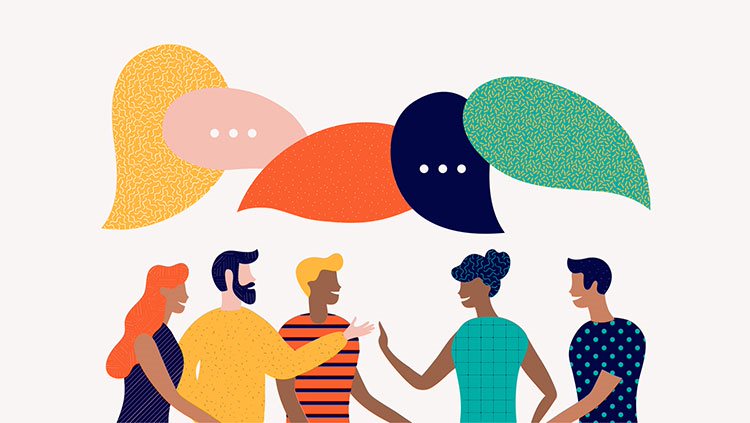
Also In Thinking & Awareness
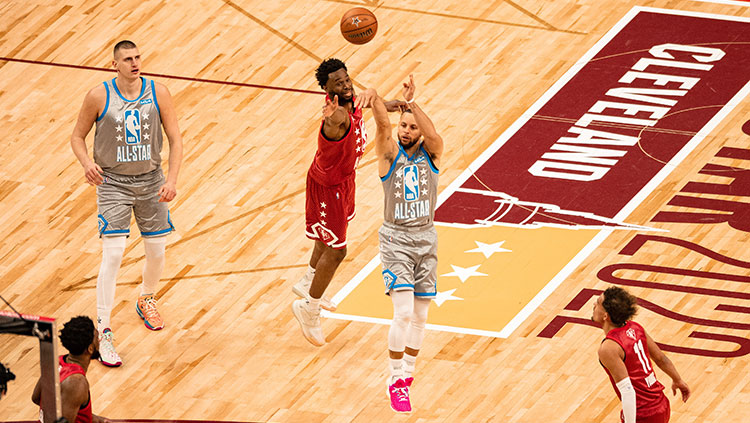
Popular articles on BrainFacts.org
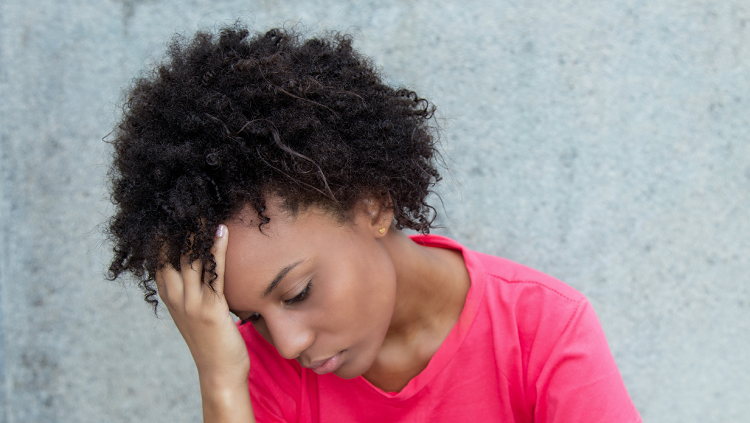
BrainFacts Book
Download a copy of the newest edition of the book, Brain Facts: A Primer on the Brain and Nervous System.
Ask An Expert
Ask a neuroscientist your questions about the brain.
Submit a Question
A beginner's guide to the brain and nervous system.
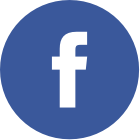
SUPPORTING PARTNERS
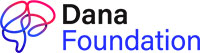
- Privacy Policy
- Accessibility Policy
- Terms and Conditions
- Manage Cookies
Some pages on this website provide links that require Adobe Reader to view.
May 15, 2024
A Cubic Millimeter of a Human Brain Has Been Mapped in Spectacular Detail
Google scientists have modeled all 150 million connections of a fragment of the human brain at nanoscale resolution
By Carissa Wong & Nature magazine
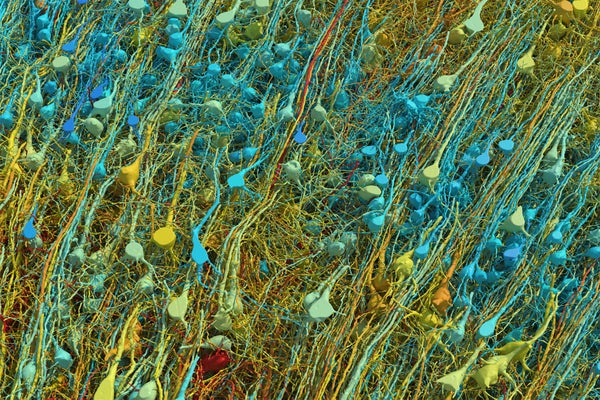
Neurons in a fragment of brain cortex.
Daniel Berger, Lichtman Lab, Harvard University
Researchers have mapped a tiny piece of the human brain in astonishing detail. The resulting cell atlas, which was described today in Science 1 and is available online , reveals new patterns of connections between brain cells called neurons, as well as cells that wrap around themselves to form knots, and pairs of neurons that are almost mirror images of each other.
The 3D map covers a volume of about one cubic millimetre, one-millionth of a whole brain, and contains roughly 57,000 cells and 150 million synapses — the connections between neurons. It incorporates a colossal 1.4 petabytes of data. “It’s a little bit humbling,” says Viren Jain, a neuroscientist at Google in Mountain View, California, and a co-author of the paper. “How are we ever going to really come to terms with all this complexity?”
Slivers of brain
On supporting science journalism.
If you're enjoying this article, consider supporting our award-winning journalism by subscribing . By purchasing a subscription you are helping to ensure the future of impactful stories about the discoveries and ideas shaping our world today.
The brain fragment was taken from a 45-year-old woman when she underwent surgery to treat her epilepsy. It came from the cortex, a part of the brain involved in learning, problem-solving and processing sensory signals. The sample was immersed in preservatives and stained with heavy metals to make the cells easier to see. Neuroscientist Jeff Lichtman at Harvard University in Cambridge, Massachusetts, and his colleagues then cut the sample into around 5,000 slices — each just 34 nanometres thick — that could be imaged using electron microscopes.
Jain’s team then built artificial-intelligence models that were able to stitch the microscope images together to reconstruct the whole sample in 3D. “I remember this moment, going into the map and looking at one individual synapse from this woman’s brain, and then zooming out into these other millions of pixels,” says Jain. “It felt sort of spiritual.”
When examining the model in detail, the researchers discovered unconventional neurons, including some that made up to 50 connections with each other. “In general, you would find a couple of connections at most between two neurons,” says Jain. Elsewhere, the model showed neurons with tendrils that formed knots around themselves. “Nobody had seen anything like this before,” Jain adds.
The team also found pairs of neurons that were near-perfect mirror images of each other. “We found two groups that would send their dendrites in two different directions, and sometimes there was a kind of mirror symmetry,” Jain says. It is unclear what role these features have in the brain.
Proofreaders needed
The map is so large that most of it has yet to be manually checked, and it could still contain errors created by the process of stitching so many images together. “Hundreds of cells have been ‘proofread’, but that’s obviously a few per cent of the 50,000 cells in there,” says Jain. He hopes that others will help to proofread parts of the map they are interested in. The team plans to produce similar maps of brain samples from other people — but a map of the entire brain is unlikely in the next few decades, he says.
“This paper is really the tour de force creation of a human cortex data set,” says Hongkui Zeng, director of the Allen Institute for Brain Science in Seattle. The vast amount of data that has been made freely accessible will “allow the community to look deeper into the micro-circuitry in the human cortex”, she adds.
Gaining a deeper understanding of how the cortex works could offer clues about how to treat some psychiatric and neurodegenerative diseases. “This map provides unprecedented details that can unveil new rules of neural connections and help to decipher the inner working of the human brain,” says Yongsoo Kim, a neuroscientist at Pennsylvania State University in Hershey.
This article is reproduced with permission and was first published on May 9, 2024 .
Scientists Imaged and Mapped a Tiny Piece of Human Brain. Here’s What They Found
With the help of an artificial intelligence algorithm, the researchers produced 1.4 million gigabytes of data from a cubic millimeter of brain tissue
/https://tf-cmsv2-smithsonianmag-media.s3.amazonaws.com/accounts/headshot/Will-Sullivan-photo.png)
Will Sullivan
Daily Correspondent
:focal(1664x1093:1665x1094)/https://tf-cmsv2-smithsonianmag-media.s3.amazonaws.com/filer_public/c7/ba/c7baacef-cb16-4351-bd03-27bd56c1b461/connectomics2024-1-excitatoryneuronsoriginal.png)
Researchers have made a digital map showing a tiny chunk of a human brain in unprecedented detail.
Based on a brain tissue sample that had been surgically removed from a person, the map represents a cubic millimeter of brain—an area about half the size of a grain of rice. But even that tiny segment is overflowing with 1.4 million gigabytes of information—containing about 57,000 cells, 230 millimeters of blood vessels and 150 million synapses, the connections between neurons.
The researchers published their findings in the journal Science on Friday. They have made the data set freely available online and provided tools for analyzing and proofreading it.
“In one respect, our data set is miniscule,” Jeff Lichtman , a co-author of the study and biologist at Harvard University, tells Popular Science ’s Lauren Leffer. “But it doesn’t feel small, because when you get in it, you see it’s like a gigantic forest.”
“It’s a little bit humbling,” Viren Jain , a co-author of the study and neuroscientist at Google, says to Nature News ’ Carissa Wong. “How are we ever going to really come to terms with all this complexity?”
The first such map of a brain was made in 1986, when researchers cataloged the 302 neurons of a roundworm, Jain writes in a Google blog post about the new research. In 2020, the Google team mapped 25,000 neurons in part of a fruit fly brain. They’ve also been involved in mapping parts of the brains of the zebra finch (a small bird) and zebrafish larvae.
For the new paper, the researchers turned to a piece of brain tissue from a person with epilepsy. To access a lesion in the patient’s hippocampus, a surgeon had removed the tissue from their left anterior temporal lobe , a part of the brain thought to play a role in our memory of objects, people, words and facts.
Scientists then used an electron microscope to image more than 5,000 slices of the tissue, each of which was only around 30 nanometers thick. The process took close to 11 months. Artificial intelligence algorithms then reconstructed the cells and their connections in 3D.
“The aim was to get a high resolution view of this most mysterious piece of biology that each of us carries around on our shoulders,” Lichtman tells the Guardian ’s Ian Sample. “The reason we haven’t done it before is that it is damn challenging. It really was enormously hard to do this.”
“It’s probably the most computer-intensive work in all of neuroscience,” Michael Hawrylycz , a computational neuroscientist at the Allen Institute for Brain Science who did not contribute to the findings, tells MIT Technology Review ’s Cassandra Willyard. “There is a Herculean amount of work involved.”
The data has already yielded some unexpected findings for the researchers. “There were just so many things in it that were incompatible with what you would read in a textbook,” Lichtman says to MIT Technology Review .
For instance, they found some rare sites where neurons were connected by more than 50 synapses. This is incredibly uncommon—more than 96 percent of connections between neurons have just one synapse, and more than 99 percent have three or fewer synapses, per Google’s blog post.
These strong connections might show “what learning looks like in the brain,” Lichtman hypothesizes to the Guardian , representing behaviors so well-practiced that they require almost no thought at all, such as moving your foot from the accelerator to the brake when stopping a car.
The team also found cases of axons, the tendrils on neurons that transmit messages, wrapping themselves into knots—but they don’t know why. “Nobody had seen anything like this before,” Jain tells Nature News .
While the tissue sample didn’t have any evidence of disease, the researchers can’t be certain whether these surprises are in some way related to the person’s epilepsy or treatments they were receiving. Studying other tissue samples could give them a clearer idea.
Next, the researchers would like to map the hippocampus of a mouse. But in the meantime, the human data they have so far will allow other researchers the chance to learn more about the human brain.
“Not only is this an impressive technological feat, this is a tool and a resource that is really aimed at sharing with the world and getting all of this scientific information out there,” Tim Mosca , a neuroscientist at Thomas Jefferson University who did not contribute to the findings, tells Popular Science . “This group has done an amazing job designing all of the new tools and the pipelines to make this available to anyone who wants to look at it, wants to think about it, wants to use this in their research.”
Get the latest stories in your inbox every weekday.
/https://tf-cmsv2-smithsonianmag-media.s3.amazonaws.com/accounts/headshot/Will-Sullivan-photo.png)
Will Sullivan | | READ MORE
Will Sullivan is a science writer based in Washington, D.C. His work has appeared in Inside Science and NOVA Next .
- Featured Experts
- Perspectives
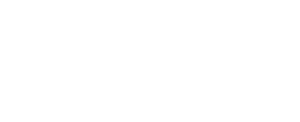
Singularity
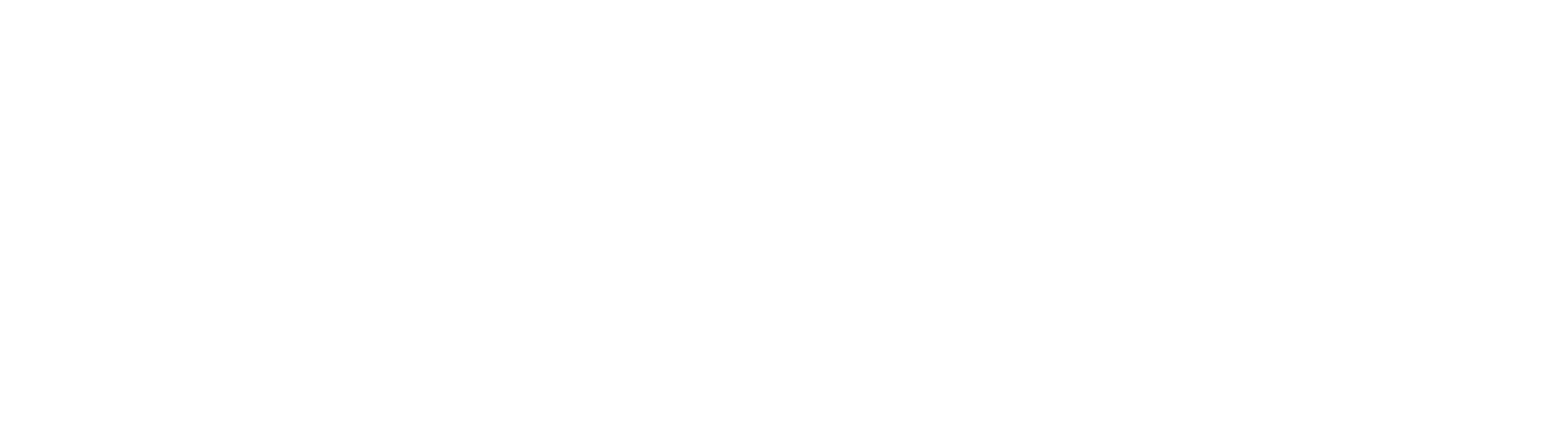
Scientists just published the most detailed map of a cubic millimeter of the human brain. Smaller than a grain of rice, the mapped section of brain includes over 57,000 cells, 230 millimeters of blood vessels, and 150 million synapses.
The project, a collaboration between Harvard and Google, is looking to accelerate connectomics—the study of how neurons are wired together—over a much larger scale.
Our brains are like a jungle.
Neuron branches crisscross regions, forming networks that process perception, memories, and even consciousness. Blood vessels tightly wrap around these branches to provide nutrients and energy. Other brain cell types form intricate connections with neurons, support the brain’s immune function, and fine-tune neural network connections.
In biology, structure determines function. Like tracing wires of a computer, mapping components of the brain and their connections can improve our understanding of how the brain works—and when and why it goes wrong. A brain map that charts the jungle inside our heads could help us tackle some of the most perplexing neurological disorders, such as Alzheimer’s disease, and decipher the origins of emotions, thoughts, and behaviors.
Aided by machine learning tools from Google Research, the Harvard team traced neurons, blood vessels, and other brain cells at nanoscale levels. The images revealed previously unknown quirks in the human brain—including mysterious tangles in neuron wiring and neurons that connect through multiple “contacts” to other cells. Overall, the dataset incorporates a massive 1.4 petabytes of information—roughly the storage amount of a thousand high-end laptops—and is free to explore .
“It’s a little bit humbling,” Dr. Viren Jain, a neuroscientist at Google and study author, told Nature . “How are we ever going to really come to terms with all this complexity?” The database, first released as a preprint paper in 2021, has already garnered much enthusiasm in the scientific field.
“It’s probably the most computer-intensive work in all of neuroscience,” Dr. Michael Hawrylycz, a computational neuroscientist at the Allen Institute for Brain Science, who was not involved in the project, told MIT Technology Review .
Why So Complicated?
Many types of brain maps exist. Some chart gene expression in brain cells; others map different cell types across the brain. But the goal is the same. They aim to help scientists understand how the brain works in health and disease .
The connectome details highways between brain regions that “talk” to each other. These connections, called synapses, number in the hundreds of trillions in human brains—on the scale of the number of stars in the universe.
Decades ago, the first whole-brain wiring map detailed all 302 neurons in the roundworm Caenorhabditis elegans . Because its genetics are largely known, the lowly worm delivered insights, such as how the brain and body communicate to increase healthy longevity. Next, scientists charted the fruit fly connectome and found the underpinnings of spatial navigation.
More recently, the MouseLight Project and MICrONS have been deciphering a small chunk of a mouse’s brain—the outermost area called the cortex. It’s hoped such work can help inform neuro-inspired AI algorithms with lower power requirements and higher efficacy.
But mice are not people. In the new study, scientists mapped a cubic millimeter of human brain tissue from the temporal cortex—a nexus that’s important for memory, emotions, and sensations. Although just one-millionth of a human brain, the effort reconstructed connections in 3D at nanoscale resolution.
Slice It Up
Sourcing is a challenge when mapping the human brain. Brain tissues rapidly deteriorate after trauma or death, which changes their wiring and chemistry. Brain organoids—”mini-brains” grown in test tubes—somewhat resemble the brain’s architecture, but they can’t replicate the real thing.
Here, the team took a tiny bit of brain tissue from a 45-year-old woman with epilepsy during surgery—the last resort for those who suffer severe seizures and don’t respond to medication.
Using a machine like a deli-meat slicer armed with a diamond knife, the Harvard team, led by connectome expert Dr. Jeff Lichtman, meticulously sliced the sample into 5,019 cross sections. Each was roughly 30 nanometers thick—a fraction of the width of a human hair. They imaged the slices with an electron microscope, capturing nanoscale cellular details, including the “factories” inside cells that produce energy, eliminate waste, or transport molecules.
Piecing these 2D images into a 3D reconstruction is a total headache. A decade ago, scientists had to do it by hand. Jain’s team at Google developed an AI to automate the job. The AI was able to track fragments of whole components—say, a part of a neuron (its body or branches)—and stick them back together throughout the images.
In total, the team pieced together thousands of neurons and over a hundred million synaptic connections. Other brain components included blood vessels and myelin—a protective molecular “sheath” covering neurons. Like electrical insulation, when myelin deteriorates, it causes multiple brain disorders.
“I remember this moment, going into the map and looking at one individual synapse from this woman’s brain, and then zooming out into these other millions of pixels,” Jain told Nature . “It felt sort of spiritual.”
A Whole New World
Even a cursory look at the data led to surprising insights into the brain’s intricate neural wiring.
Cortical neurons have a forest-like structure for input and a single “cable” that delivers output signals. Called axons, these are dotted with thousands of synapses connecting to other cells.
Usually, a synapse grabs onto just one spot of a neighboring neuron. But the new map found a rare, strange group that connects with up to 50 points. “We’ve always had a theory that there would be super connections, if you will, amongst certain cells…But it’s something we’ve never had the resolution to prove,” Dr. Tim Mosca, who was not involved in the work, told Popular Science . These could be extra-potent connections that allow neural communications to go into “autopilot mode,” like when riding a bike or navigating familiar neighborhoods.
More strange structures included “axon whorls” that wrapped around themselves like tangled headphones. An axon’s main purpose is to reach out and connect with other neurons—so why do some fold into themselves? Do they serve a purpose, or are they just a hiccup in brain wiring? It’s a mystery. Another strange observation found pairs of neurons that perfectly mirrored each other. What this symmetry does for the brain is also unknown.
The bottom line: Our understanding of the brain’s connections and inner workings is still only scratching the surface. The new database is a breakthrough, but it’s not perfect. The results are from a single person with epilepsy, which can’t represent everyone. Some wiring changes, for example, may be due to the disorder. The team is planning a follow-up to separate epilepsy-related circuits from those that are more universal in people.
Meanwhile, they’ve opened the entire database for anyone to explore. And the team is also working with scientists to manually examine the results and eliminate potential AI-induced errors during reconstruction. So far, hundreds of cells have been “proofread” and validated by humans, but it’s just a fraction of the 50,000 neurons in the database.
The technology can also be used for other species, such as the zebrafish—another animal model often used in neuroscience research—and eventually the entire mouse brain.
Although this study only traced a tiny nugget of the human brain, the atlas is a stunning way to peek inside its seemingly chaotic wiring and make sense of things. “Further studies using this resource may bring valuable insights into the mysteries of the human brain,” wrote the team.
Image Credit: Google Research and Lichtman Lab
- Neuroscience
A Massive Study Is Revealing Why Exercise Is So Good for Our Health
The crucial building blocks of life on earth form more easily in outer space, scientists create atomically thin gold with century-old japanese knife making technique, this week’s awesome tech stories from around the web (through may 18), smelting steel with sunlight: new solar trap tech could help decarbonize industrial heat, scientists step toward quantum internet with experiment under the streets of boston.
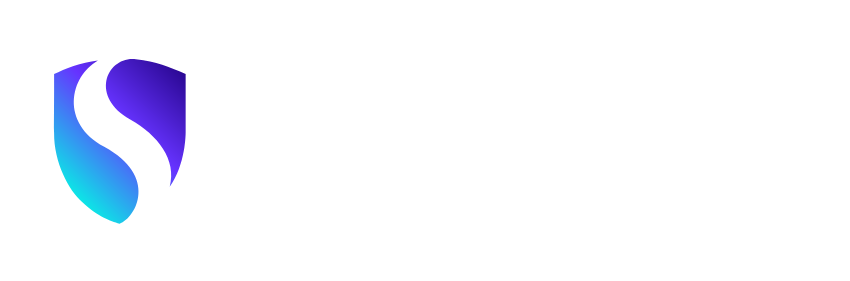
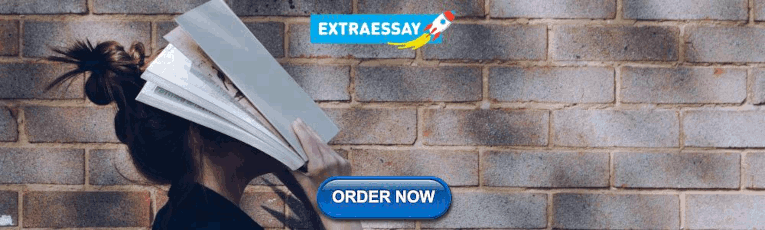
IMAGES
VIDEO
COMMENTS
In doing so, they become stored in different brain regions and new neural connections are formed that may assist problem solving. On waking, you may have formed associations between information ...
The brain is a unique organ that is responsible for many functions such as problem-solving, thinking, emotions, controlling physical movements, and mediating the perception and responses related to the five senses. The many nerve cells of the brain communicate with each other to control this activity. Each area of the brain has one or more ...
The cerebrum (front of brain) comprises gray matter (the cerebral cortex) and white matter at its center. The largest part of the brain, the cerebrum initiates and coordinates movement and regulates temperature. Other areas of the cerebrum enable speech, judgment, thinking and reasoning, problem-solving, emotions and learning.
The four lobes of the brain are regions of the cerebrum: Frontal Lobe. Location: This is the anterior or front part of the brain. Functions: Decision making, problem solving, control of purposeful behaviors, consciousness, and emotions. Parietal Lobe. Location: Sits behind the frontal lobe.
Special areas of note within this lobe are the motor cortex, the prefrontal cortex and Broca's area. Your motor cortex is responsible for body movement. Your prefrontal cortex is in charge of "executive functions," such as thinking and problem-solving. It also supervises and directs other areas of your brain.
The frontal lobe is the brain's largest region, located behind the forehead, at the front of the brain. These lobes are part of the cerebral cortex and are the largest brain structure. The frontal lobe's main functions are typically associated with 'higher' cognitive functions, including decision-making, problem-solving, thought, and ...
More than two-thirds of this layer is folded into grooves. The grooves increase the brain's surface area, allowing for inclusion of many more neurons. ... The frontal lobe is responsible for initiating and coordinating motor movements; higher cognitive skills, such as problem solving, thinking, planning, and organizing; and for many aspects ...
The brain is the human body's control system, and is part of the central nervous system (CNS). ... problem-solving ; ... It is made up of three major areas: the cerebrum, cerebellum, and brain stem.
This is the front of the brain. It is made up of the right and left hemispheres, which are joined by the corpus callosum. The cerebrum controls: initiation of movement, coordination of movement, temperature, touch, vision, hearing, judgment, reasoning, problem solving, emotions, and learning.
The second probe into problem-solving focused on the anterior cingulate cortex (ACC), a region in the front of the brain tied to functions such as decision making, conflict monitoring and reward ...
The brainstem is located at the base of the brain. This area connects the cerebrum and the cerebellum to the spinal cord, acting as a relay station for these areas. ... Their main functions are associated with higher cognitive functions, including problem-solving, decision-making, attention, intelligence, and voluntary behaviors.
The cerebral cortex is the part of the brain responsible for higher cognitive abilities. ... such as decision making, motivation, attention, learning, memory, problem-solving, and conceptual thinking. This article will discuss the anatomy and ... Posterior to the primary auditory area in the superior temporal gyrus, lies the auditory ...
The brain is a very busy organ. It is the control center for the body. It runs your organs such as your heart and lungs. It is also busy working with other parts of your body. All of your senses - sight, smell, hearing, touch, and taste - depend on your brain. Tasting food with the sensors on your tongue is only possible if the signals from ...
Based on signals from the inner ears and the muscles, the cerebellum enables the body to maintain balance and posture. The brain stem, situated at the bottom of the brain, is made up of three main ...
Controlling complex intellectual processes, such as speech, thought, concentration, problem-solving, and planning for the future. ... Many functions of the brain are performed by several areas of the brain working together (networks), not by a single area in the brain. Damage to these networks can cause the following:
Another area of the brain vital to problem solving is the prefrontal cortex, located toward the front of the brain. For a long time, it was thought that some parts of the prefrontal cortex were ...
The brain regulates an array of functions necessary to survival: the action of our five senses, the continuous monitoring of the spatial surround, contraction and relaxation of the digestive muscles, the rhythms of breathing and a regular heartbeat. As the vital functions maintain their steady course without our conscious exertion, we are accustomed to consider the brain as preeminently the ...
Yes, it is possible for a person to survive without a prefrontal cortex. However, not having this portion of the brain will have an enormous impact on their ability to reason, plan ahead, control behavior, and solve problems. A person's ability to have social relationships would also be affected severely.
Pictures of the brain in action show that adolescents' brains work differently than adults when they make decisions or solve problems. Their actions are guided more by the emotional and reactive amygdala and less by the thoughtful, logical frontal cortex. Research has also shown that exposure to drugs and alcohol during the teen years can ...
The orbitofrontal cortex, a region of the PFC located just behind the eyes, also appears to be important in affective decision-making, especially in situations involving reward and punishment. The area has been implicated in addiction as well as social behavior. Adapted from the 8th edition of Brain Facts by Marissa Fessenden.
The brain fragment was taken from a 45-year-old woman when she underwent surgery to treat her epilepsy. It came from the cortex, a part of the brain involved in learning, problem-solving and ...
Based on a brain tissue sample that had been surgically removed from a person, the map represents a cubic millimeter of brain—an area about half the size of a grain of rice. But even that tiny ...
Brain organoids—"mini-brains" grown in test tubes—somewhat resemble the brain's architecture, but they can't replicate the real thing. Here, the team took a tiny bit of brain tissue from a 45-year-old woman with epilepsy during surgery—the last resort for those who suffer severe seizures and don't respond to medication.