ORIGINAL RESEARCH article
Photosynthetic physiology of blue, green, and red light: light intensity effects and underlying mechanisms.
- Horticultural Physiology Laboratory, Department of Horticulture, University of Georgia, Athens, GA, United States
Red and blue light are traditionally believed to have a higher quantum yield of CO 2 assimilation ( QY , moles of CO 2 assimilated per mole of photons) than green light, because green light is absorbed less efficiently. However, because of its lower absorptance, green light can penetrate deeper and excite chlorophyll deeper in leaves. We hypothesized that, at high photosynthetic photon flux density ( PPFD ), green light may achieve higher QY and net CO 2 assimilation rate ( A n ) than red or blue light, because of its more uniform absorption throughtout leaves. To test the interactive effects of PPFD and light spectrum on photosynthesis, we measured leaf A n of “Green Tower” lettuce ( Lactuca sativa ) under red, blue, and green light, and combinations of those at PPFD s from 30 to 1,300 μmol⋅m –2 ⋅s –1 . The electron transport rates ( J ) and the maximum Rubisco carboxylation rate ( V c,max ) at low (200 μmol⋅m –2 ⋅s –1 ) and high PPFD (1,000 μmol⋅m –2 ⋅s –1 ) were estimated from photosynthetic CO 2 response curves. Both QY m,inc (maximum QY on incident PPFD basis) and J at low PPFD were higher under red light than under blue and green light. Factoring in light absorption, QY m,abs (the maximum QY on absorbed PPFD basis) under green and red light were both higher than under blue light, indicating that the low QY m,inc under green light was due to lower absorptance, while absorbed blue photons were used inherently least efficiently. At high PPFD , the QY inc [gross CO 2 assimilation ( A g )/incident PPFD ] and J under red and green light were similar, and higher than under blue light, confirming our hypothesis. V c,max may not limit photosynthesis at a PPFD of 200 μmol m –2 s –1 and was largely unaffected by light spectrum at 1,000 μmol⋅m –2 ⋅s –1 . A g and J under different spectra were positively correlated, suggesting that the interactive effect between light spectrum and PPFD on photosynthesis was due to effects on J . No interaction between the three colors of light was detected. In summary, at low PPFD , green light had the lowest photosynthetic efficiency because of its low absorptance. Contrary, at high PPFD , QY inc under green light was among the highest, likely resulting from more uniform distribution of green light in leaves.
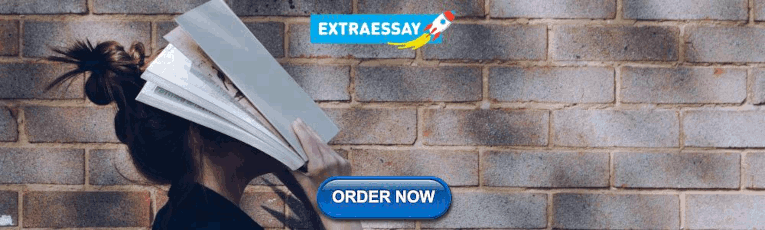
Introduction
The photosynthetic activity of light is wavelength dependent. Based on McCree’s work ( McCree, 1971 , 1972 ), photosynthetically active radiation is typically defined as light with a wavelength range from 400 to 700 nm. Light with a wavelength shorter than 400 nm or longer than 700 nm was considered as unimportant for photosynthesis, due to its low quantum yield of CO 2 assimilation, when applied as a single waveband ( Figure 1 ). Within the 400–700 nm range, McCree (1971) showed that light in the red region (600–700 nm) resulted in the highest quantum yield of CO 2 assimilation of plants. Light in the green region (500–600 nm) generally resulted in a slightly higher quantum yield than light in the blue region (400–500 nm) ( Figure 1 ; McCree, 1971 ). The low absorptance of green light is partly responsible for its low quantum yield of CO 2 assimilation. Within the visible spectrum, green leaves have the highest absorptance in the blue region, followed by red. Green light is least absorbed by green leaves, which gives leaves their green appearance ( McCree, 1971 ; Zhen et al., 2019 ).
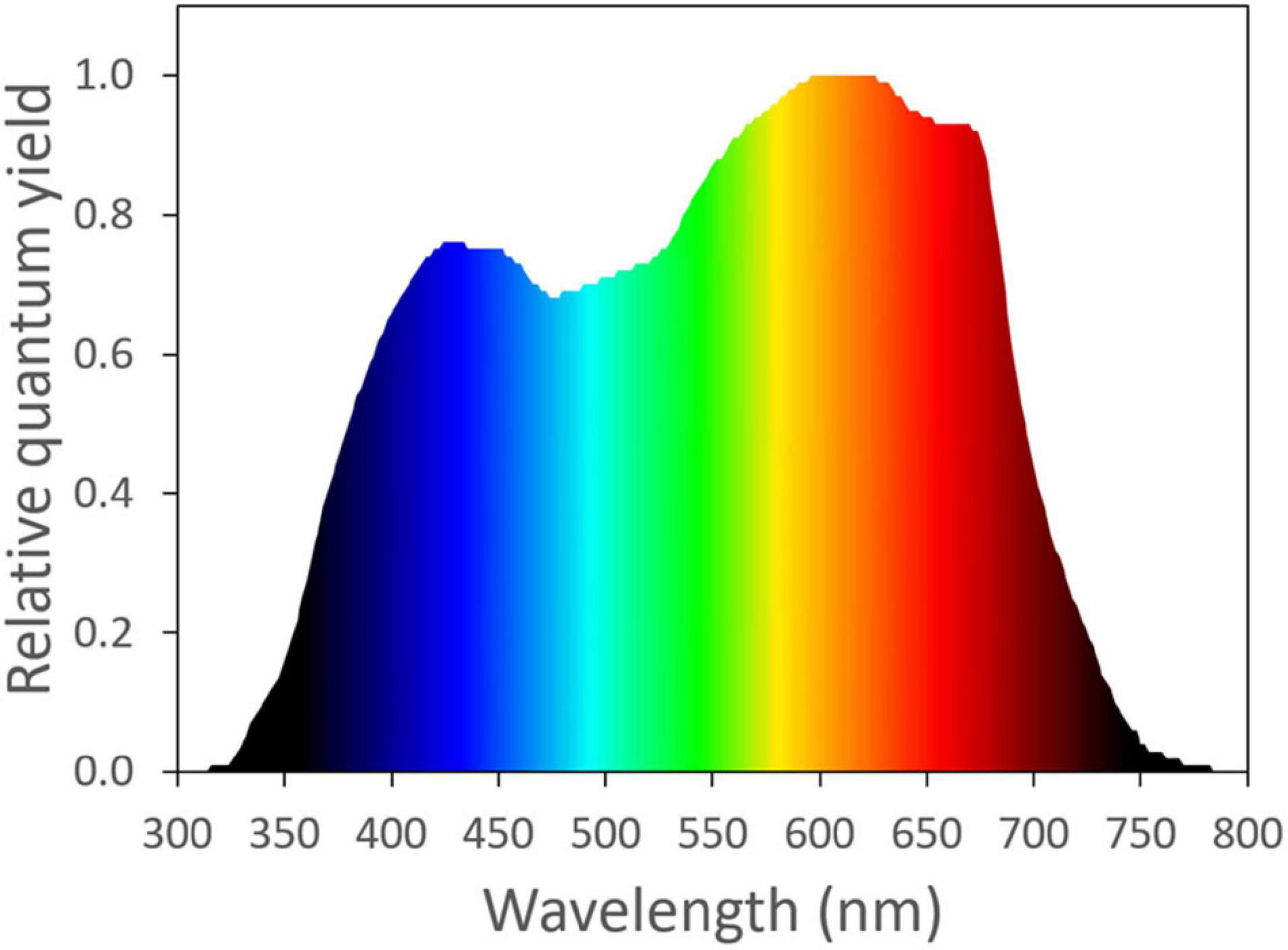
Figure 1. The normalized action spectrum of the maximum quantum yield of CO 2 assimilation for narrow wavebands of light from ultra-violet to far-red wavelengths ( McCree, 1971 ). Redrawn using data from Sager et al. (1988).
Since red and blue light are absorbed more strongly by photosynthetic pigments than green light, they are predominantly absorbed by the top few cell layers, while green light can penetrate deeper into leaf tissues ( Nishio, 2000 ; Vogelmann and Evans, 2002 ; Terashima et al., 2009 ; Brodersen and Vogelmann, 2010 ), thus giving it the potential to excite photosystems in deeper cell layers. Leaf photosynthesis may benefit from the more uniform light distribution throughout a leaf under green light. Absorption of photons by chloroplasts near the adaxial surface may induce heat dissipation of excess excitation energy in those chloroplasts, while chloroplasts deeper into the leaf receive little excitation energy ( Sun et al., 1998 ; Nishio, 2000 ). Blue and red photons, therefore, may be used less efficiently and are more likely to be dissipated as heat than green photons.
The misconception that red and blue light are used more efficiently by plants than green light still occasionally appears ( Singh et al., 2015 ), often citing McCree’s action spectrum or the poor absorption of green light by chlorophyll extracts. The limitations of McCree’s action spectrum were explained in his original paper: the quantum yield was measured under low photosynthetic photon flux density ( PPFD ), using narrow waveband light, and expressed on an incident light basis ( McCree, 1971 ), but these limitations are sometimes ignored. The importance of green light for photosynthesis has been well established in more recent studies ( Sun et al., 1998 ; Nishio, 2000 ; Terashima et al., 2009 ; Hogewoning et al., 2012 ; Smith et al., 2017 ).
From those studies, one trend has emerged that has not received much attention: there is an interactive effect of light quality and intensity on photosynthesis ( Sun et al., 1998 ; Evans and Vogelmann, 2003 ; Terashima et al., 2009 ). At low PPFD , green light has the lowest QY inc (quantum yield of CO 2 assimilation on incident light basis) because of its low absorptance; at high PPFD , on the other hand, red and blue light have a lower QY inc than green light, because of their high absorptance by photosynthetic pigments, which shifts much of the light absorption closer to the upper leaf surface. This reduces both the quantum yield of CO 2 assimilation in cells in the upper part of a leaf and light availability in the bottom part of a leaf.
The interactive effect between light quality and intensity was illustrated in an elegant study that quantified the differential quantum yield, or the increase in leaf CO 2 assimilation per unit of additional light ( Terashima et al., 2009 ). The differential quantum yield was measured by adding red or green light to a background illumination of white light of different intensities. At low background white light levels, the differential quantum yield of red light was higher than that of green light, due to the low absorptance of green light. But as the background light level increased, the differential quantum yield of green light decreased more slowly than that of red light, and was eventually higher than that of red light ( Terashima et al., 2009 ). The red light was absorbed efficiently by the chloroplasts in the upper part of leaves. With a high background level of white light, those chloroplasts already received a large amount of excitation energy from white light and up-regulated non-photochemical quenching (NPQ) to dissipate excess excitation energy as heat, causing the additional red light to be used inefficiently. Green light, on the other hand, was able to reach the chloroplasts deeper in the mesophyll and excited those chloroplasts that received relatively little excitation energy from white light. Therefore, with high background white light intensity, additional green light increased leaf photosynthesis more efficiently than red light ( Terashima et al., 2009 ).
In this paper, we present a comprehensive study to explore potential interactive effect of light intensity and light quality on C 3 photosynthesis and underlying processes. We quantified the photosynthetic response of plants to blue, green, and red light over a wide PPFD range to better describe how light intensity and waveband interact. In addition, we examined potential interactions among blue, green, and red light, using light with different ratios and intensities of the three narrow waveband lights. To get a better understanding of the biochemical reasons for the effects of light spectrum and intensity on CO 2 assimilation, we constructed assimilation – internal leaf CO 2 ( C i ) response curves ( A/C i curves) under blue, green, and red light, as well as combinations of the three narrow waveband lights at both high and low PPFD . We hypothesized that effects of different light spectra would be reflected in the electron transport rate ( J ) required to regenerate consumed ribulose 1,5-bisphosphate (RuBP), rather than the maximum carboxylation rate of ribulose-1,5-bisphosphate carboxylase/oxygenase (Rubisco) ( V c,max ).
Materials and Methods
Plant material.
Lettuce “Green Towers” plants were grown from seed in 1.7 L round pots filled with soilless substrate (Fafard 4P Mix, Sun Gro Horticulture, Agawam, MA, United States). The plants were grown in a growth chamber (E15, Conviron, Winnipeg, Manitoba, Canada) at 23.2 ± 0.8°C (mean ± SD), under white fluorescent light with a 14-hr photoperiod, vapor pressure deficit (VPD) of 1.20 ± 0.43 kPa and a PPFD of 200–230 μmol⋅m –2 ⋅s –1 at the floor level, and ambient CO 2 concentration. Plants were sub-irrigated when necessary with a nutrient solution containing 100 mg⋅L –1 N, made with a complete, water-soluble fertilizer (Peter’s Excel 15-5-15 Cal-Mag fertilizer, Everris, Marysville, OH, United States).
Leaf Absorptance, Transmittance, and Reflectance
Leaf absorptance was determined using a method similar to that of Zhen et al. (2019) . Three plants were randomly selected. A newly expanded leaf from each plant was illuminated with a broad-spectrum halogen bulb (70W; Sylvania, Wilmington, MA, United States) for leaf transmittance measurement. Transmittance was measured with a spectroradiometer (SS-110, Apogee, Logan, UT, United States). The halogen light spectrum was taken as reference measurement with the spectroradiometer placed directly under the halogen bulb in a dark room. Then, a lettuce leaf was placed between the halogen bulb and spectroradiometer, with its adaxial side facing the halogen bulb and transmitted light was measured. Leaf transmittance was then calculated on 1 nm resolution. Light reflectance of the leaves was measured using a spectrometer with a leaf clip (UniSpec, PP systems, Amesbury, MA, United States). Light absorptance was calculated as 1− r e f l e c t a n c e − t r a n s m i t t a n c e . We verified that this method results in similar absorptance spectra as the use of an integrating sphere. Absorptance of each of the nine light spectra used in this study were calculated from the overall leaf absorptance spectrum and the spectra of the red, green, and blue LEDs.
Leaf Photosynthesis Measurements
All gas exchange measurements were made with a leaf gas exchange system (CIRAS-3, PP Systems). Light was provided by the LEDs built into the chlorophyll fluorescence module (CFM-3, PP Systems). This module has dimmable LED arrays of different colors, with peaks at 653 nm [red, full width at half maximum (FWHM) of 17 nm], 523 nm (green, FWHM of 36 nm), and 446 nm (blue, FWHM of 16 nm). Nine different combinations of red, green, and blue light were used in this study ( Table 1 ). Throughout the measurements, the environmental conditions inside the cuvette were controlled by the leaf gas exchange system. Leaf temperature was 23.0 ± 0.1°C, CO 2 concentration was 400.5 ± 4.1 μmol⋅mol –1 , and the VPD of air in the leaf cuvette was 1.8 ± 0.3 kPa (mean ± SD).
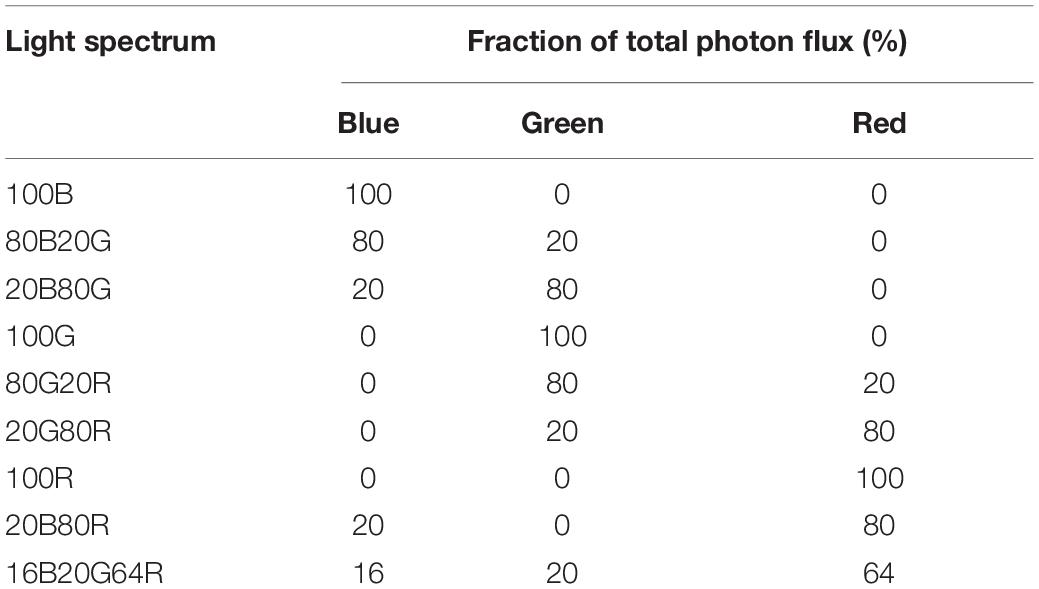
Table 1. List of light spectrum abbreviations and their spectral composition.
Photosynthesis – Light Response Curves
To explore photosynthetic efficiency of light with different spectra, we constructed light response curves for lettuce plants using each light spectrum. Lettuce plants were exposed to 10 PPFD levels ranging from 30 to 1,300 μmol⋅m –2 ⋅s –1 (30, 60, 90, 120, 200, 350, 500, 700, 1,000, and 1,300 μmol⋅m –2 ⋅s –1 ) in ascending orders for light response curves. Photosynthetic measurements were taken on 40–66 days old lettuce plants. Lettuce plants were taken out of the growth chamber and dark-adapted for 30 min. Starting from the lowest PPFD , one newly expanded leaf was exposed to all nine spectra. Net CO 2 assimilation rate ( A n ) of the leaf was measured using the leaf gas exchange system. Under each light spectrum, three A n readings were recorded at 10 s intervals after readings were stable (about 4–20 min depending on PPFD after changing PPFD and spectrum). The three A n readings were averaged for analysis. After A n measurements under all nine light spectra were taken, the leaf was exposed to the next PPFD level and A n measurements were taken with the light spectra in the same order, until measurements were completed at all PPFD levels. Throughout the light response curves, C i decreased with increasing PPFD , from 396 ± 10 μmol⋅mol –1 at a PPFD of 30 μmol⋅m –2 ⋅s –1 to 242 ± 44 μmol⋅mol –1 at a PPFD of 1,300 μmol⋅m –2 ⋅s –1 . To account for the potential effect of plants and the order of the spectra on assimilation rates, the order of the different spectra was re-randomized for each light response curve, using a Latin square design with plant and spectrum as the blocking factors. Data were collected on nine different plants.
Regression curves (exponential rise to maximum) were fitted to the data for each light spectrum and replication (plant):
where R d is the dark respiration rate, QY m,inc is the maximum quantum yield of CO 2 assimilation (initial slope of light response curve, mol of CO 2 fixed per mol of incident photons) and A g,max is the light-saturated gross assimilation rate. The A n,max is the light-saturated net assimilation rate and was calculated as A n , m a x = A g , m a x - R d . The maximum quantum yield of CO 2 assimilation was also calculated on absorbed light basis as Q Y m , a b s = Q Y m , i n c l i g h t a b s o r p t a n c e .
The instantaneous quantum yield of CO 2 assimilation based on incident PPFD ( QY inc ) was calculated as A g P P F D for each PPFD at which A n was measured, where the gross CO 2 assimilation rate ( A g ) was calculated as A g = A n + R d . To account for differences in absorptance among the different light spectra, the quantum yield of CO 2 assimilation was also calculated based on absorbed light base, as Q Y a b s = A g P P F D × l i g h t a b s o r p t a n c e , where light absorptance is the absorptance of lettuce leaves for each specific light spectrum. The differential QY , the increase in assimilation rate per unit of additional incident PPFD , was calculated as the derivative of Eq. 1:
Photosynthesis – Internal CO 2 Response ( A/C i ) Curves
To explore the underlying physiological mechanisms of assimilation responses to different light spectra, we constructed A/C i curves. Typically, A/C i curves are collected under saturating PPFD . We collected A/C i curves at two PPFD s (200 and 1,000 μmol⋅m –2 ⋅s –1 ) to explore interactive effects of light spectrum and PPFD on the assimilation rate. At a PPFD of 200 μmol⋅m –2 ⋅s –1 , red light has the highest A n and green light the lowest A n , while at PPFD of 1,000 μmol⋅m –2 ⋅s –1 , red and green light resulted in the highest A n and blue light in the lowest A n .
We used the rapid A/C i response (RACiR) technique that greatly accelerates the process of constructing A/C i curves ( Stinziano et al., 2017 ). We used a Latin square design, similar to the light response curves. A/C i curves were measured under the same nine spectra used for the light response curves. Nine lettuce plants were used as replicates. For each A/C i curve, CO 2 concentration in the leaf cuvette started from 0 μmol⋅mol –1 , steadily ramping to 1,200 μmol⋅mol –1 over 6 min. A reference measurement was also taken at the beginning of each replication with an empty cuvette to correct for the reaction time of the leaf gas exchange system. Post-ramp data processing was used to calculate the real A and C i with the spreadsheet provided by PP systems, which yielded the actual A/C i curves with C i range of about 100–950 μmol mol –1 . Throughout the data collection, leaf temperature was 24.4 ± 1.3°C and VPD in the cuvette was 1.4 ± 0.2 kPa.
Curve fitting for A/C i curves was done by minimizing the residual sum of squares, following the protocol developed by Sharkey et al. (2007) . Among our nine replicates, four plants did not show clear Rubisco limitations at low PPFD and for those plants Rubisco limitation ( V c,ma x ) was not included in the model ( Sharkey et al., 2007 ). We therefore report V c,max values for high PPFD only. The J was determined for all light spectra at both PPFD s. We therefore report V c,max was determined for all light spectra only at high PPFD . The quantum yield of electron transport [ QY(J) ] was calculated on both incident and absorbed PPFD basis as Q Y ( J ) i n c = J P P F D and Q Y ( J ) a b s = Q Y ( J ) i n c l i g h t a b s o r p t a n c e , respectively. We did not estimate triose phosphate utilization, because the A/C i curves often did not show a clear plateau.
Data Analysis
The QY m,inc , QY m,abs , and A g,max were analyzed with ANOVA to determine the effects of light spectrum using SAS (SAS University Edition; SAS Institute, Cary, NC, United States). A n , QY inc , and QY abs at each PPFD level and V c,max and J estimated from A/C i curves were similarly analyzed with ANOVA using SAS. A n at different PPFD levels were analyzed with regression analysis to detect interactive effect of blue, green, and red light on leaf assimilation rates using the fractions of red, blue, and green light as explanatory variables (JMP Pro 15, SAS Institute).
Leaf Absorptance
A representative spectrum of light absorptance, reflectance and transmittance of a newly fully expanded lettuce leaf is shown in Figure 2 . In the blue region, 400–500 nm, the absorptance by “Green Towers” lettuce leaves was high and fairly constant, averaging 91.6%. The leaf absorptance decreased as the wavelength increased from 500 to 551 nm where the absorptance minimum was 69.8%. Absorptance increased again at longer wavelengths, with a second peak at 666 nm (92.6%). Above 675 nm, the absorptance decreased steadily to <5% at 747 nm ( Figure 2 ). The absorptance spectrum of our lettuce leaves is similar to what McCree (1971) obtained for growth chamber-grown lettuce, with the exception of slightly higher absorptance in the green part of the spectrum in our lettuce plants. Using this spectrum, the absorptance of the blue, green, and red LED lights were calculated to be 93.2 ± 1.0%, 81.1 ± 1.9% and 91.6 ± 1.1%, respectively. Absorptance of all nine spectra was calculated based on their ratios of red, green, and blue light ( Table 2 ).
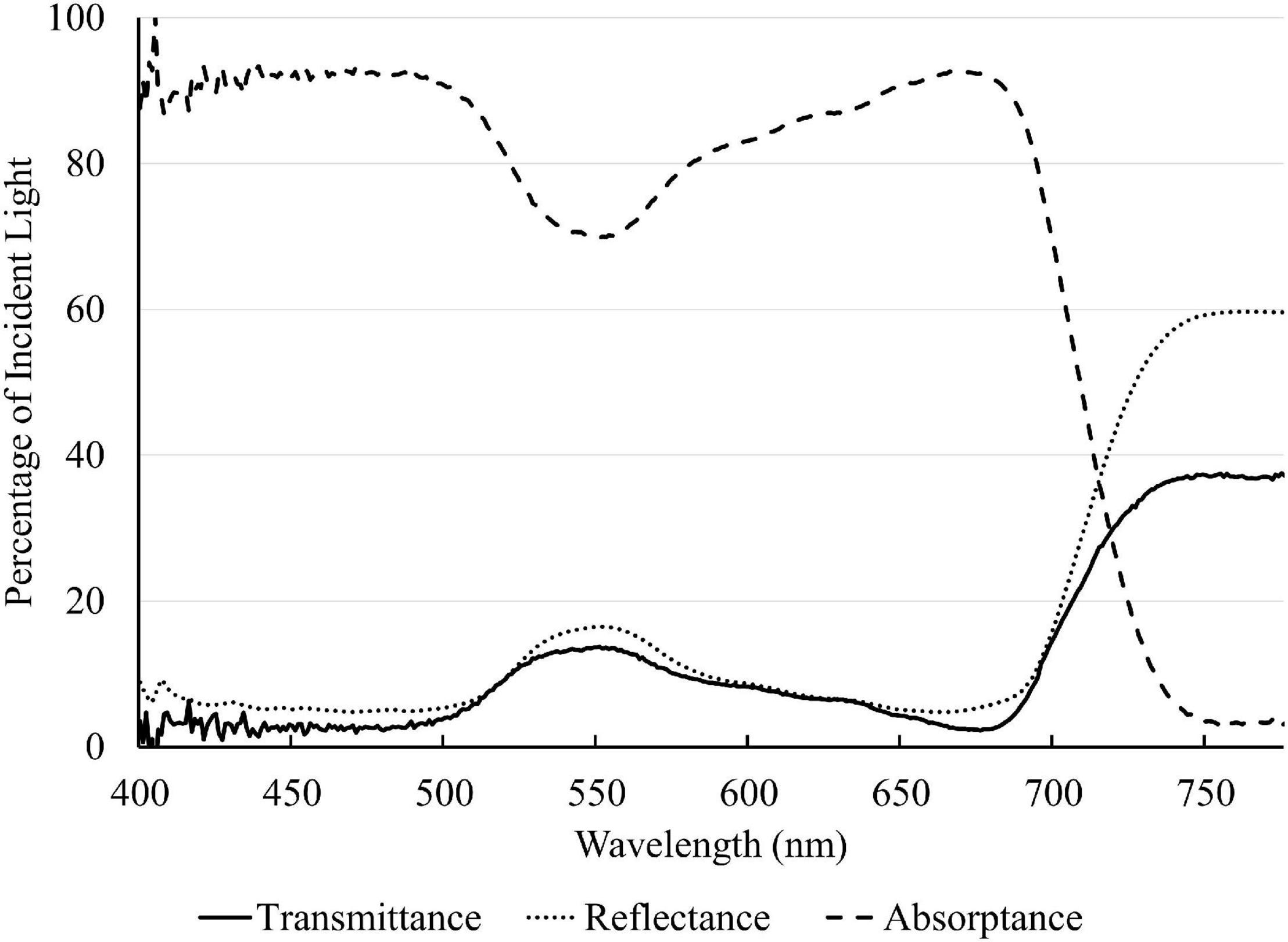
Figure 2. Light absorptance, reflectance, and transmittance spectrum of a newly fully expanded “Green Towers” lettuce leaf.
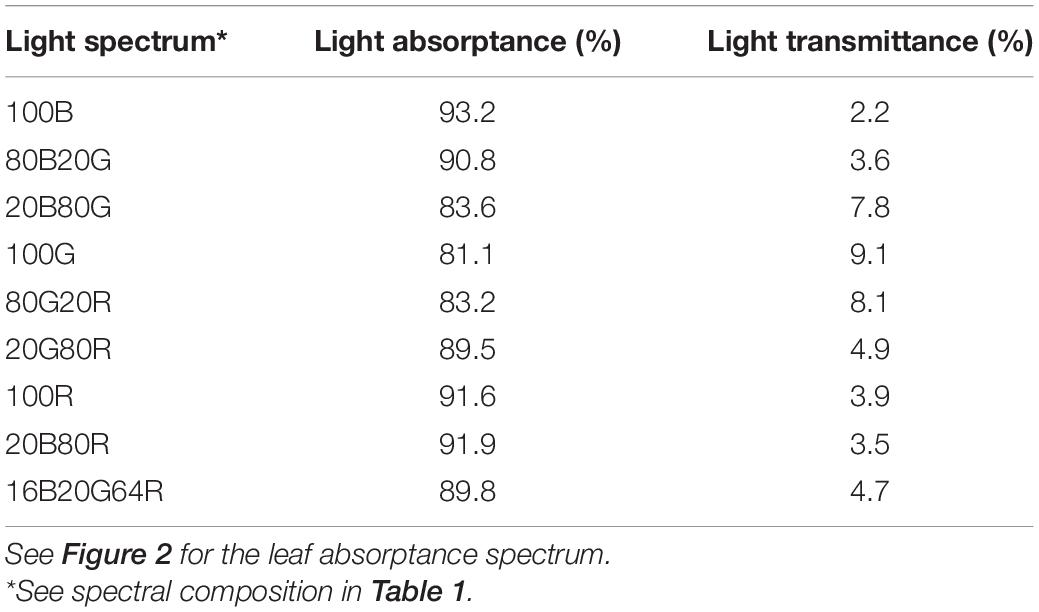
Table 2. Light absorptance and transmittance of new fully expanded “Green towers” lettuce leaves under nine light spectra.
Light Quality and Intensity Effects on Photosynthetic Parameters
Light response curves of lettuce under all nine spectra are shown in Figure 3 , with regression coefficients in Supplementary Table 1 . It is worth noting that a few plants showed photoinhibition under 100B (decrease in A n with PPFD > 1,000 μmol⋅m –2 ⋅s –1 ). Those data were excluded in curve fitting for light response curves to better estimate asymptotes. Photoinhibition was not observed under other spectra.
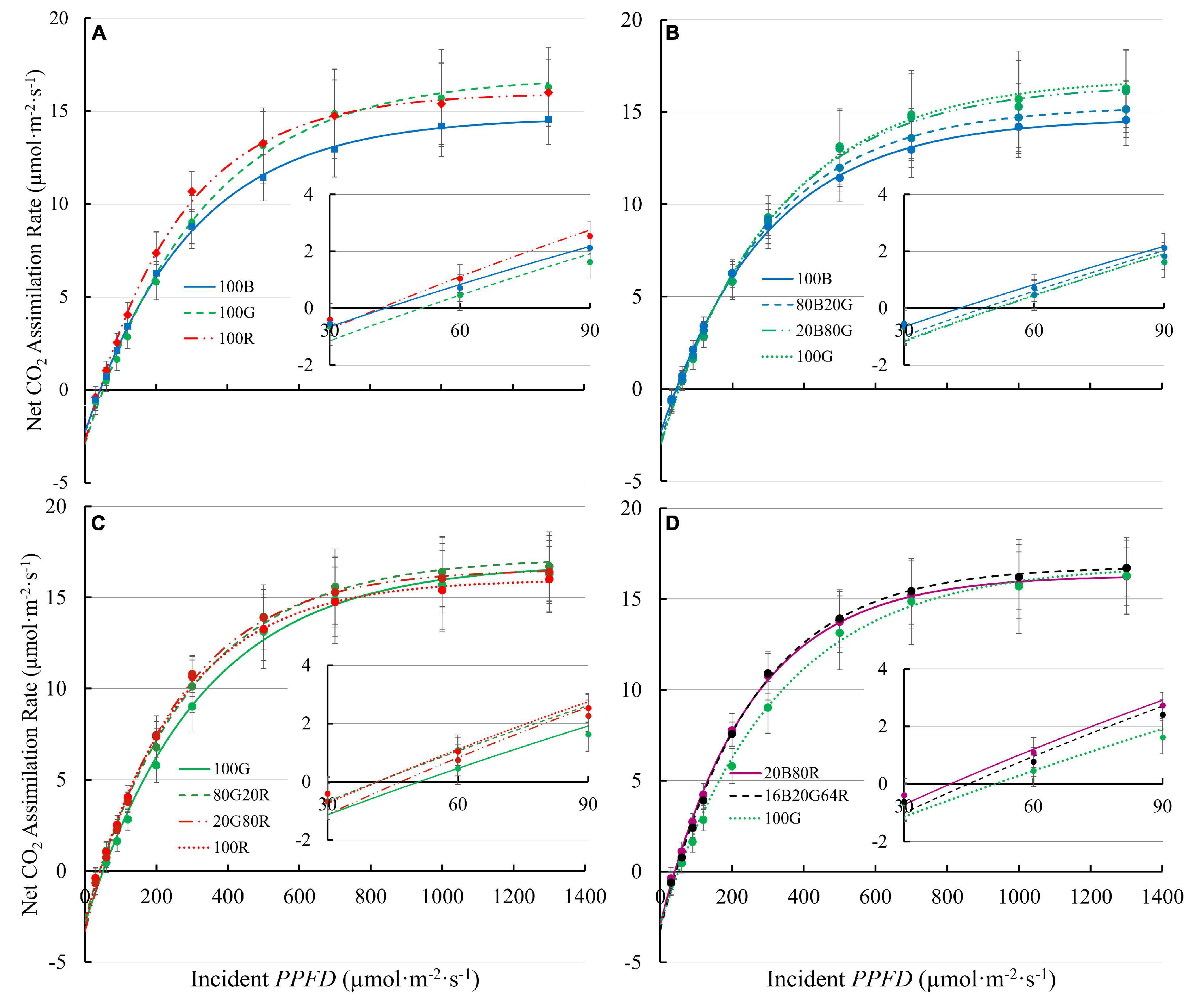
Figure 3. Net assimilation ( A n ) – light response curves of “Green Towers” lettuce under nine light spectra. Error bars represent the standard deviation ( n = 9). Inserts show A n against PPFD of 30-90 μmol⋅m –2 ⋅s –1 s to better show the initial slopes of curves. The composition of the nine light spectra is shown in Table 1 . The light spectra in the graphs are (A) 100B, 100G, and 100R; (B) 100B, 80B20G, 20B80G, and 100G; (C) 100G, 80G20R, 20G80R, and 100R; and (D) 20B80R, 16B20G64R, and 100G.
The QY m,inc of lettuce plants was 22 and 27% higher under red light (74.3 mmol⋅mol –1 ) than under either 100G (60.8 mmol⋅mol –1 ) or 100B (58.4 mmol⋅mol –1 ), respectively ( Figure 4A and Supplementary Table 1 ). Spectra with a high fraction of red light (64% or more) resulted in a high QY m,inc ( Figure 4A ), while 80G20R resulted in an intermediate QY m,inc ( Figure 4A ). To determine whether differences in QY m,inc were due to differences in absorptance or in the ability of plants to use the absorbed photons for CO 2 assimilation, we also calculated QY m,abs . On an absorbed light basis, 100B light still resulted in the lowest QY m,abs (62.7 mmol⋅mol –1 ) and red light resulted in the highest QY m,abs (81.1 mmol⋅mol –1 ) among narrow waveband lights ( Figure 4B ). Green light resulted in a QY m,abs (74.9 mmol⋅mol –1 ) similar to that under red light, but significantly higher than that of blue light ( Figure 4B ). We did not find any interactions (synergism or antagonism) between lights of different colors, with all physiological responses under mixed spectra being similar to the weighted average of responses under single colors. Thus, for the rest of the results we focus on the three narrow waveband spectra.
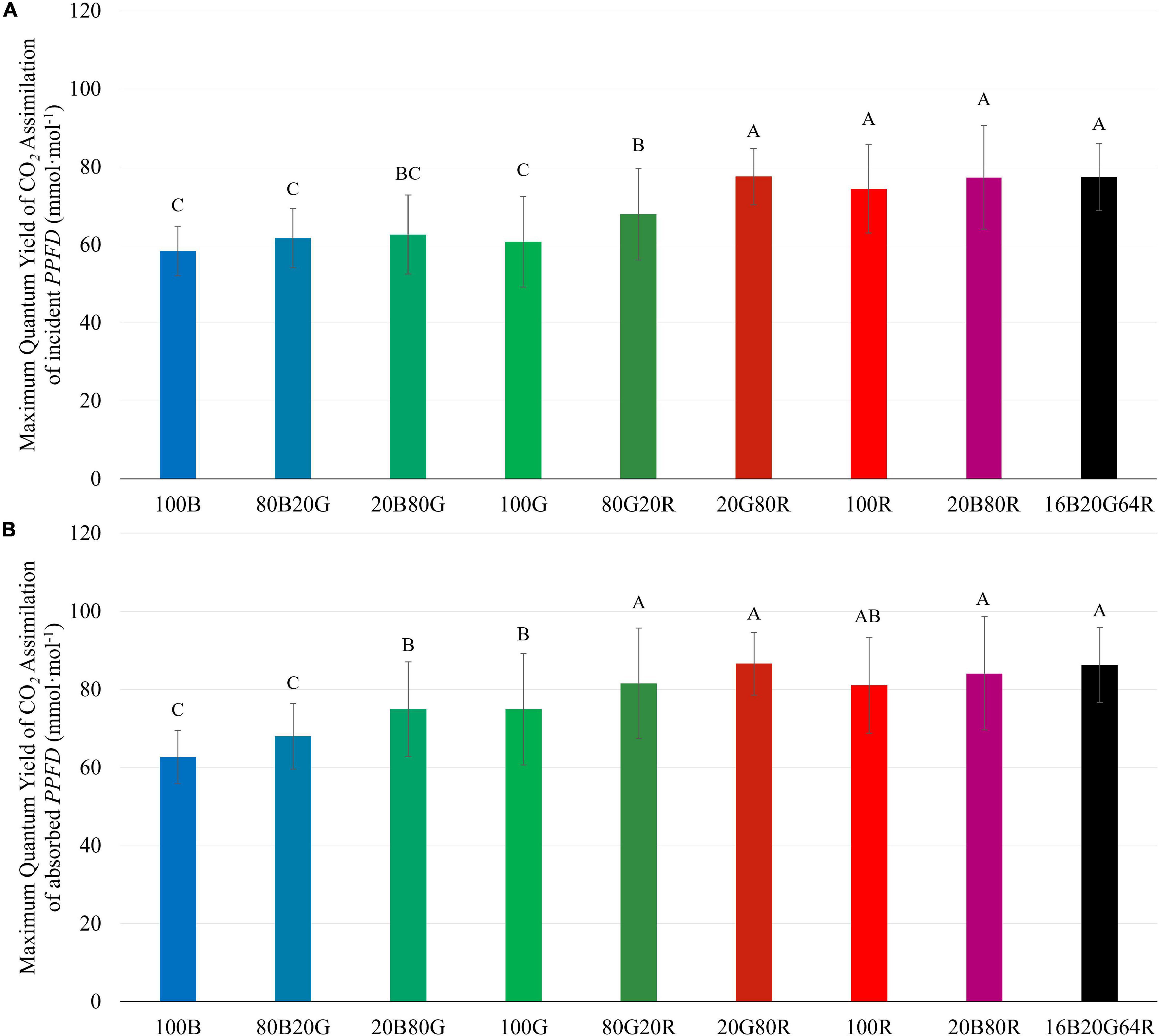
Figure 4. Maximum quantum yield of CO 2 assimilation of “Green Towers” lettuce based on incident ( QY m,inc ) (A) and absorbed light ( QY m,abs ) (B) under nine different light spectra. Values are calculated as the initial slope of the light response curves of corresponding light spectra (see Figure 3 ). Bars with the same letter are not statistically different ( p ≤ 0.05). Error bars represent the standard deviation ( n = 9). The composition of the nine light spectra is shown in Table 1 .
Among the three narrow waveband lights, 100G resulted in the highest A g,max (20.0 μmol⋅m –2 ⋅s –1 ), followed by red (18.9 μmol⋅m –2 ⋅s –1 ), and blue light (17.0 μmol⋅m –2 ⋅s –1 ) ( Figure 5 and Supplementary Table 1 ). As with QY m ,inc and QY m,abs , combining two or three colors of light resulted in an A g,max similar to the weighted averages of individual light colors.
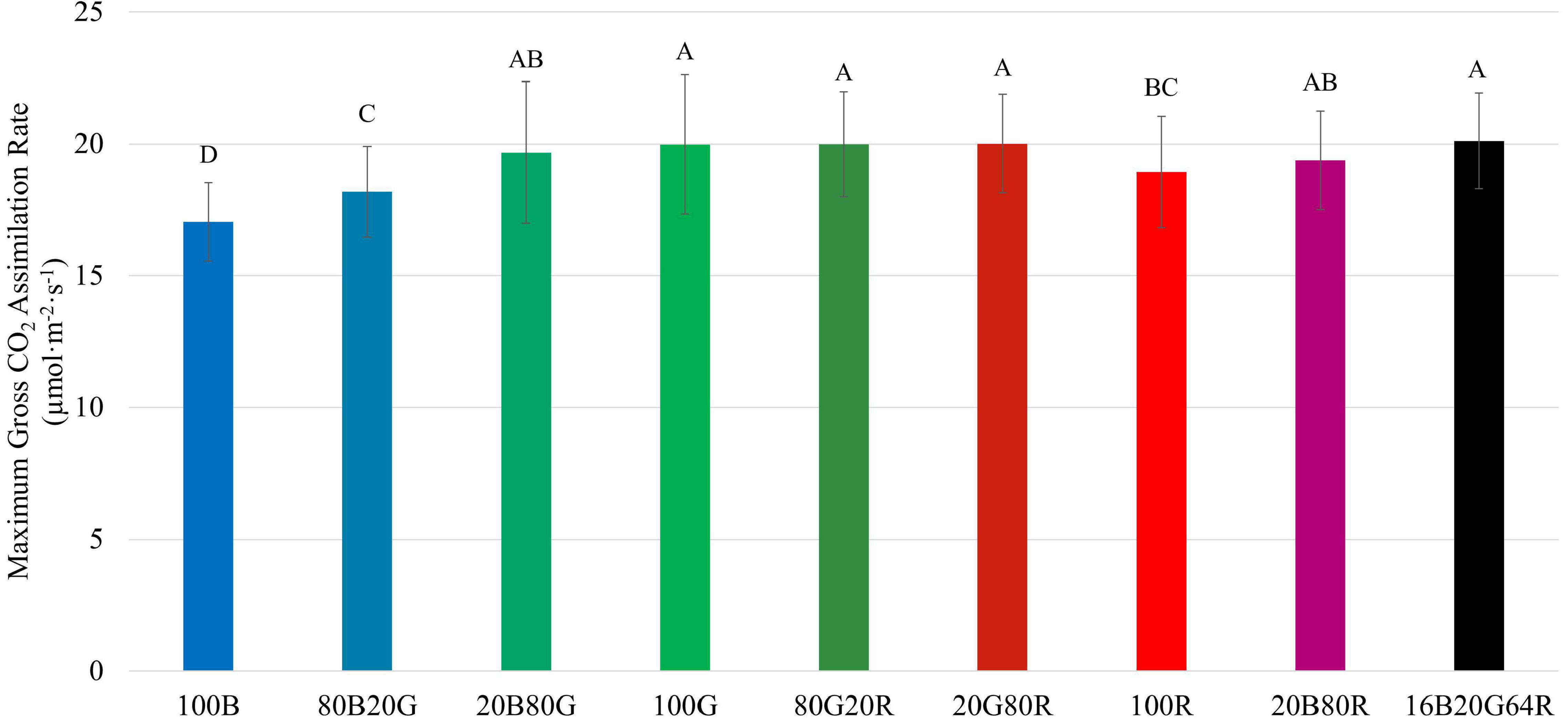
Figure 5. Maximum gross assimilation rate ( A g,max ) of “Green Towers” lettuce under different light spectra, calculated from the light response curves. Bars with the same letter are not statistically different ( p ≤ 0.05). Error bars represent standard deviation ( n = 9). The composition of the nine light spectra is shown in Table 1 .
QY inc initially increased with increasing PPFD and peaked at 90–200 μmol⋅m –2 ⋅s –1 , then decreased at higher PPFDs ( Figure 6A ). The QY inc under 100R was higher than under either green or blue light at low PPFD (≤300 μmol⋅m –2 ⋅s –1 ). Although 100G resulted in lower QY inc than 100B at low PPFD (≤300 μmol⋅m –2 ⋅s –1 ), the decrease in QY inc under 100G with increasing PPFD was slower than that with 100B or 100R. Above 500 μmol m –2 s –1 , the QY inc with 100G was comparable to the QY inc with 100R, and higher than with 100B ( Figure 6A ). The QY abs with 100R was higher than that with either 100G or 100B at PPFDs from 60 to 120 μmol⋅m –2 ⋅s –1 ( p < 0.05). The QY abs with 100G was similar to 100B at low PPFD , but decreased slower than that with either 100R or 100B as PPFD increased. At PPFD ≥ 500 μmol⋅m –2 ⋅s –1 , QY abs was lowest under 100B among the three monochromatic lights ( p < 0.05) ( Figure 6B ).
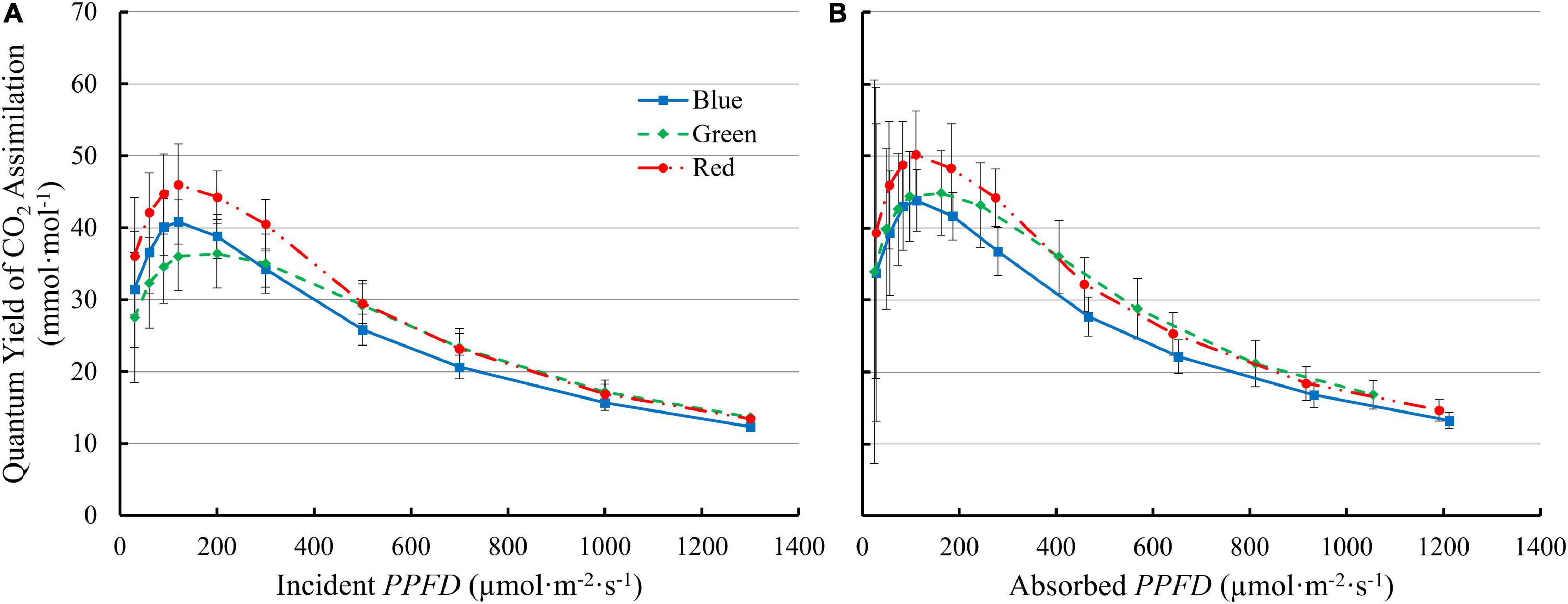
Figure 6. The quantum yield of CO 2 assimilation of “Green Towers” lettuce as a function of incident ( QY inc ) (A) and absorbed PPFD ( QY abs ) (B) under blue, green, and red LED light. Error bars represent the standard deviation ( n = 9).
The differential QY , which quantifies the increase in CO 2 assimilation per unit of additional PPFD , decreased with increasing PPFD . The differential QY with 100R was higher than those with 100B and 100G at low PPFD . At a PPFD of 30 μmol⋅m –2 ⋅s –1 , the differential QY was 70.5 mmol⋅mol –1 for 100R, 59.4 mmol⋅mol –1 for 100G, and 55.8 mmol⋅mol –1 for 100B ( Figure 7 ). However, the differential QY with 100R decreased rapidly with increasing PPFD and was lower than the differential QY with 100G at high PPFD ( Figure 7 ). At high PPFD , the differential QY with 100G was highest among three monochromatic light ( Figure 7 ). For instance, at a PPFD of 1,300 μmol⋅m –2 ⋅s –1 , the differential QY with 100G was 1.09 mmol⋅mol –1 , while those with 100B and 100R were 0.64 mmol⋅mol –1 and 0.46 mmol⋅mol –1 , respectively ( Figure 7 ).
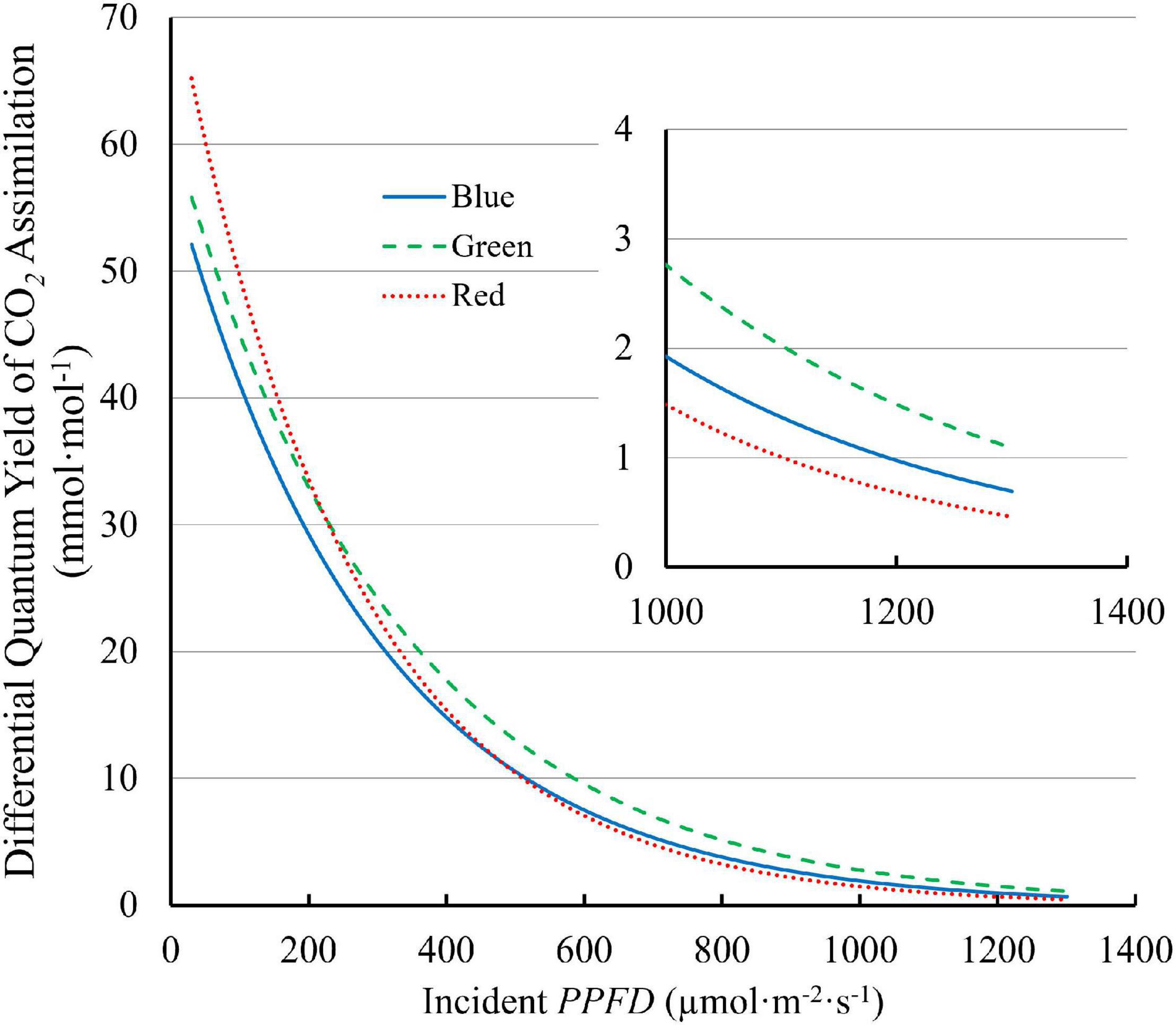
Figure 7. The differential quantum yield of CO 2 assimilation ( differential QY ) of “Green Towers” lettuce under blue, green, and red LED light as a function of the PPFD . The differential QY is the increase in net assimilation per unit additional PPFD and was calculated as the first derivate of the light response curves ( Figure 3 ). The insert shows the differential quantum yield plotted at PPFDs of 1,000–1,300 μmol m –2 s –1 s to better show differences at high PPFD (note the different y -axis scale).
Effect of Light Spectrum and Intensity on J and V c,max
J of lettuce leaves at low PPFD was lowest under 100G (47.4 μmol⋅m –2 ⋅s –1 ), followed by 100B (56.1 μmol⋅m –2 ⋅s –1 ), and highest under 100R (64.1 μmol⋅m –2 ⋅s –1 ) ( Figure 8A ). At high PPFD , on the other hand, J of leaves exposed to 100G (115.3 μmol⋅m –2 ⋅s –1 ) and 100R (112.1 μmol⋅m –2 ⋅s –1 ) were among the highest, while J of leaves under 100B was the lowest (97.0 μmol⋅m –2 ⋅s –1 ) ( Figure 8A ). At high PPFD , V c,max of leaves under blue light (59.3 μmol⋅m –2 ⋅s –1 ) was lower than V c,max of leaves under 16B20G64R light (72.1 μmol⋅m –2 ⋅s –1 ), but none of the other treatments differed significantly ( Figure 8 ). When PPFD increased from 200 to 1,000 μmol⋅m –2 ⋅s –1 , J under green light increased by 143%, while J under blue and red light increased by 73% and 75%, respectively ( Figure 8A ). J and V c,max at high PPFD were strongly correlated ( R 2 = 0.82) ( Supplementary Figure 3 ).
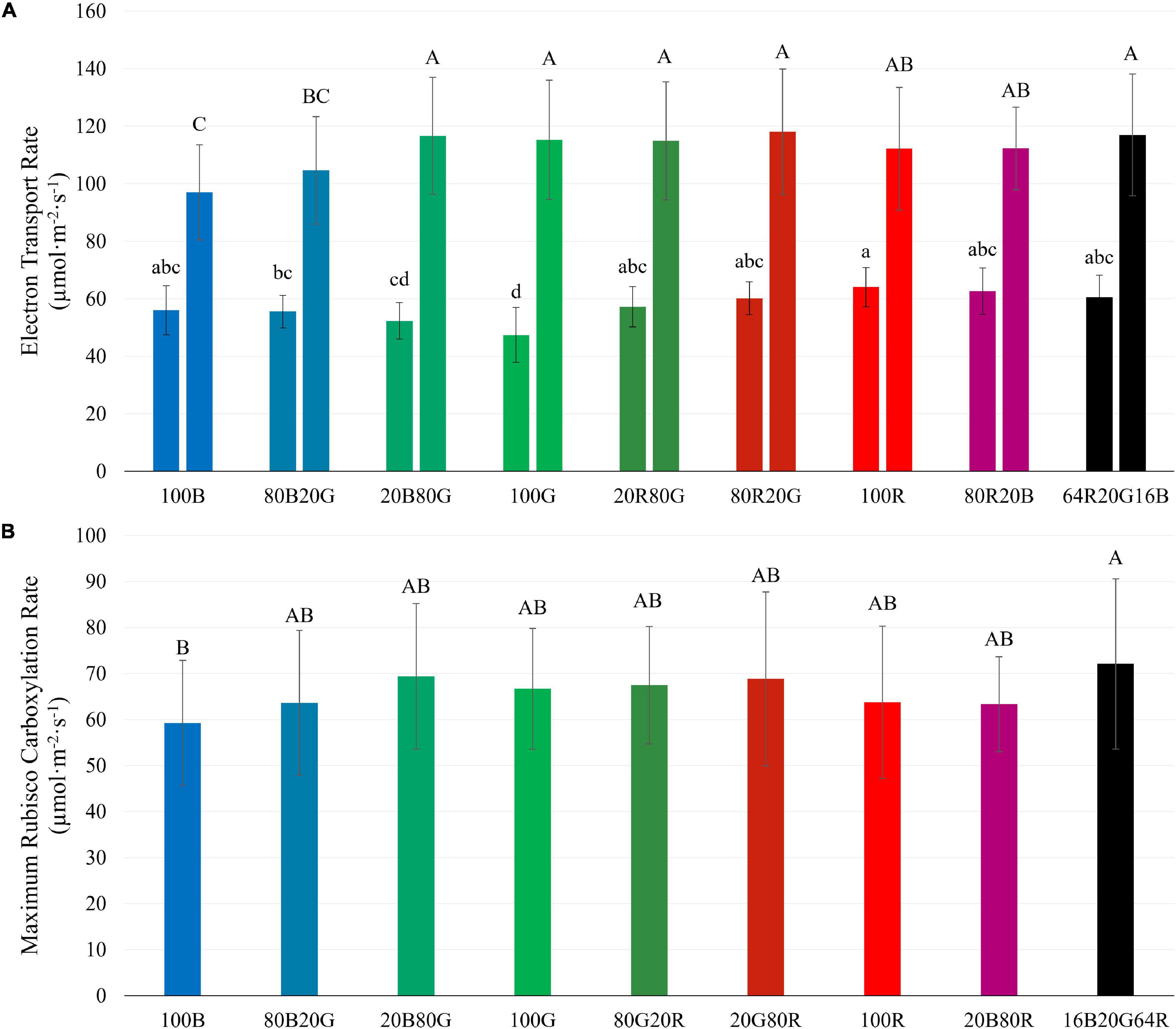
Figure 8. Electron transport rate ( J ) at PPFD s of 200 (left bars) and 1,000 μmol m –2 s –1 (right bars) (A) and maximum Rubisco carboxylation rate ( V c,max ) at a PPFD of 1,000 μmol m –2 s –1 (B) of “Green Towers” lettuce, as estimated from A/C i curves under different light spectra. Bars with the same letter are not statistically different ( p ≤ 0.05). Error bars represent the standard deviation ( n = 9). The light composition of the nine light spectra is shown in Table 1 .
Interactive Effect of Light Spectrum and PPFD on Photosynthesis
There was an interactive effect of light spectrum and PPFD on photosynthetic properties of lettuce. Under low light conditions (≤200 μmol⋅m –2 ⋅s –1 ), the QY inc of lettuce leaves under green light was lowest among blue, green, and red light ( Figure 6A ), due to its lower absorptance by lettuce leaves. After accounting for absorptance, green photons were used at similar efficiency as blue photons, while red photons were used most efficiently ( Figure 6B ). The QY m,abs under green and red light were higher than under blue light ( Figure 4B ). At high PPFD , green and red light had similar quantum yield, higher than that of blue light, both on an absorbed and incident light basis ( Figure 6A ). Multiple factors contributed to the interactive effect of light spectrum and PPFD on quantum yield and photosynthesis.
Light Absorptance and Non-Photosynthetic Pigments Determine Assimilation at Low PPFD
QY m,inc with blue and green light was lower than with red light ( Figure 4A ), consistent with McCree’s action spectrum ( McCree, 1971 ). But when taking leaf absorptance into account, QY m,abs was similar under green and red light and lower under blue light ( Figure 4B ). Similarly, at low PPFD (≤200 μmol⋅m –2 ⋅s –1 ), QY inc of lettuce leaves was highest under red, intermediate under blue, and lowest under green light. When accounting for leaf absorptance, QY abs under red light remained highest and QY abs under both green and blue light were similar at low PPFD ( Figure 6A ). Consistent with our data, previous studies also documented that, once absorbed, green light can drive photosynthesis efficiently at low PPFD ( Balegh and Biddulph, 1970 ; McCree, 1971 ; Evans, 1987 ; Sun et al., 1998 ; Nishio, 2000 ; Terashima et al., 2009 ; Hogewoning et al., 2012 ; Vogelmann and Gorton, 2014 ). For example, the QY m,abs of spinach ( Spinacia oleracea ) and cabbage ( Brassica oleracea L. ) was highest under red light, followed by that under green light and lowest with blue light. But on incident light basis, QY m,inc of under green light was lower than under red or blue light ( Sun et al., 1998 ).
Both our data ( Figure 4B ) and those of Sun et al. (1998) show that QY m,abs with blue light is lower than that with red and green light, indicating that blue light is used intrinsically less efficiently by lettuce. Blue light, and, to a lesser extent, green light is absorbed not just by chlorophyll, but also by flavonoids and carotenoids ( Sun et al., 1998 ). Those pigments can divert energy away from photochemistry and thus reduce the QY abs under blue light. Flavonoids (e.g., anthocyanins) are primarily located in the vacuole and cannot transfer absorbed light energy to photosynthetic pigments ( Sun et al., 1998 ). Likewise, free carotenoids do not contribute to photochemistry ( Hogewoning et al., 2012 ). Carotenoids in light-harvesting antennae and reaction centers channel light energy to photochemistry, but with lower transfer efficiency than chlorophylls ( Croce et al., 2001 ; de Weerd et al., 2003a , b ; Wientjes et al., 2011 ; Hogewoning et al., 2012 ). Therefore, absorption of blue light by flavonoids and carotenoids reduces the quantum yield of CO 2 assimilation. Thus, even with the high absorptance of blue light by green leaves, QY m,abs of leaves under blue light was the lowest among the three monochromatic lights ( Figure 4B ). It is likely that the lower QY abs under green light than that under red light was also due to absorption of green light by carotenoids and flavonoids ( Hogewoning et al., 2012 ). At high PPFD , absorption of blue light by flavonoids and carotenoids still occurs, but this is less of a limiting factor for photosynthesis, since light availability is not limiting under high PPFD .
Light Dependence of Respiration and Rubisco Activity May Reduce the Quantum Yield at Low PPFD
At PPFD s below 200 μmol⋅m –2 ⋅s –1 , the QY inc and QY abs of lettuce showed an unexpected pattern in response to PPFD ( Figure 6 ). Unlike the quantum yield of PSII, which decreases exponentially with increasing PPFD ( Weaver and van Iersel, 2019 ), QY inc and QY abs increased initially with increasing PPFD ( Figure 6 ). A similar pattern was previously observed by Craver et al. (2020) in petunia ( Petunia × hybrida ) seedlings. This pattern could result from light-dependent regulation of respiration ( Croce et al., 2001 ), alternative electron sinks such as nitrate reduction ( Skillman, 2008 ; Nunes-Nesi et al., 2010 ), or Rubisco activity ( Campbell and Ogren, 1992 ; Zhang and Portis, 1999 ). In our calculations, we assumed that the leaf respiration in the light was the same as R d . However, leaf respiration in the light is lower than in the dark, in a PPFD -dependent manner ( Brooks and Farquhar, 1985 ; Atkin et al., 1997 ), which can lead to overestimation of A g with increasing PPFD . When we accounted for this down-regulation of respiration, using the model by Müller et al. (2005) to correct A g , QY inc , and QY abs , we found that depression of respiration by light did not explain the initial increase in QY inc and QY abs we observed ( Supplementary Figure 4 ). Alternative electron sinks in the chloroplasts that are upregulated in response to light can explain the low QY inc , and QY abs at low PPFD , because they compete with the Calvin cycle for reducing power (ferredoxin/NADPH). Such processes include photorespiration ( Krall and Edwards, 1992 ), nitrate assimilation ( Nunes-Nesi et al., 2010 ), sulfate assimilation ( Takahashi et al., 2011 ) and the Mehler reaction ( Badger et al., 2000 ) and their effect on QY inc , and QY abs would be especially notable under low PPFD ( Supplementary Figure 5 ).
Upregulation of Rubisco activity by Rubisco activase in the light may also have contributed to the increase in QY inc and QY abs at low PPFD ( Campbell and Ogren, 1992 ; Zhang and Portis, 1999 ). In the dark, 2-carboxy-D-arabinitol-1-phosphate (CA1P) or RuBP binds strongly to the active sites of Rubisco, preventing carboxylation activity. In the light, Rubisco activase releases the inhibitory CA1P or RuBP from the catalytic site of Rubisco, in a light-dependent manner ( Campbell and Ogren, 1992 ; Zhang and Portis, 1999 ; Parry et al., 2008 ). At PPFD < 120 μmol⋅m –2 ⋅s –1 , low Rubisco activity may have limited photosynthesis.
Light Distribution Within Leaves Affects QY at High PPFD
Except for the initial increase at low PPFD , both QY inc and QY abs decreased with increasing PPFD . QY inc decreased slower under green than under red or blue light ( Figure 6A ). At a PPFD ≥ 500 μmol⋅m –2 ⋅s –1 , QY inc under green light was higher than that under blue light ( Figure 6A ). Accordingly, A n under blue light was lower than under green and red light at PPFD s above 500 μmol⋅m –2 ⋅s –1 ( Figure 3A ). The lower QY inc under blue light than under green and red light at high PPFD can be explained by disparities in the light distribution within leaves.
Blue and red light were strongly absorbed by lettuce leaves (93.2 and 91.6%, respectively), while green light was absorbed less (81.1%) ( Table 2 ). Similar low green absorptance was found in sunflower ( Helianthus annuus L.), snapdragon ( Antirrhínum majus L.) ( Brodersen and Vogelmann, 2010 ), and spinach ( Vogelmann and Han, 2000 ). In leaves of those species, absorption of red and blue light peaked in the upper 20% of leaves, and declined sharply further into the leaf. Absorption of red light decreased slower with increasing depth than that of blue light ( Vogelmann and Han, 2000 ; Brodersen and Vogelmann, 2010 ). Green light absorption peaked deeper into leaves, and was more evenly distributed throughout leaves, because of low absorption of green light by chlorophyll ( Vogelmann and Han, 2000 ; Brodersen and Vogelmann, 2010 ). The more even distribution of green light within leaves, as compared to red and blue light, can explain the interactive effects between PPFD and light spectrum on leaf photosynthesis. It was estimated that less than 10% of blue light traveled through the palisade mesophyll and reached the spongy mesophyll in spinach, while about 35% of green light and 25% of red light did so ( Vogelmann and Evans, 2002 ). It was also estimated that chlorophyll in the lowermost chloroplasts of spinach leaves absorbed about 10% of green and <2% of blue light, compared to chlorophyll in the uppermost chloroplasts ( Vogelmann and Evans, 2002 ; Terashima et al., 2009 ).
The more uniform green light distribution within leaves may be a key contributor to higher leaf level QY inc under high PPFD because less heat dissipation of excess light energy is needed ( Nishio, 2000 ; Terashima et al., 2009 ). Reaction centers near the adaxial leaf surface receive more excitation energy under blue, and to a lesser extent under red light, than under green light, because of the differences in absorptance. Consequently, under high intensity blue light, NPQ is up-regulated in the chloroplasts near the adaxial leaf surface to dissipate some of the excitation energy ( Sun et al., 1998 ; Nishio, 2000 ), lowering the QY inc under blue light. Since less green light is absorbed near the adaxial surface, less heat dissipation is required. When incident light increased from 150 to 600 μmol⋅m –2 ⋅s –1 , the fraction of whole leaf CO 2 assimilation that occurred in the top half of spinach leaves remained the same under green light (58%), but decreased from 87 to 73% under blue light. This indicates more upregulation of heat dissipation in the top of the leaves under blue, than under green light ( Evans and Vogelmann, 2003 ). On the other hand, the bottom half of the leaves can still utilize the available light with relatively high QY inc , since the amount of light reaching the bottom half is relatively low, even under high PPFD ( Nishio, 2000 ). By channeling more light to the under-utilized bottom part of leaves, leaves could achieve higher QY inc even under high intensity green light. In our study, high QY inc under green light and low QY inc under blue light at high PPFD ( Figure 6 ) can be thus explained by the large disparities in the light environment in chloroplasts from the adaxial to the abaxial side of leaves due to differences in leaf absorptance. Similarly, differential QY of lettuce leaves was highest under green light and lower under blue and red light at high PPFD (>300 μmol⋅m –2 ⋅s –1 ) ( Figure 7 ), also potentially because of the more uniform distribution of green light and the uneven distribution of blue and red light in leaves.
Along the same line, A n of lettuce leaves was the lowest under blue light at PPFD > 500 μmol⋅m –2 ⋅s –1 ( Figure 3 ). Also, A n of lettuce leaves approached light saturation at lower PPFD s under blue and red light, than under green light ( Figure 3A ). Under blue, green, and red light, lettuce leaves reached 95% of A n,max at PPFD s of 954, 1,110 and 856 μmol⋅m –2 ⋅s –1 , respectively. This can be seen more clearly in the differential QY at high PPFD ( Figure 7 ). At a PPFD of 1,300 μmol⋅m –2 ⋅s –1 , green light had a differential QY of 1.09 mmol⋅mol –1 , while that of red and blue light was only 0.46 and 0.69 mmol⋅mol –1 , respectively ( Figure 7 ). Green light also resulted in a higher A g,max (22.9 μmol⋅m –2 ⋅s –1 ) than red and blue light (21.8 and 19.3 μmol⋅m –2 ⋅s –1 , respectively) ( Figure 5 ). As discussed before, the high A g,max under green light resulted from the more uniform light distribution under green light, allowing deeper cell layers to photosynthesize more. Previous research similarly found that at high PPFD (>500 μmol⋅m –2 ⋅s –1 ), A n of both spinach and cabbage were lower under blue light than under white, red and green light ( Sun et al., 1998 ). Overall, under high PPFD , the differences in light distribution throughout a leaf are important to quantum yield and assimilation rate, since it affects NPQ up-regulation ( Sun et al., 1998 ; Nishio, 2000 ). However, light distribution within a leaf is less important at low than at high PPFD , because upregulation of NPQ increases with increasing PPFD ( Zhen and van Iersel, 2017 ).
Light Spectrum Affects J , but Not V c,max
We examined the effect of light quality and intensity on J and V c,max ( Figure 8 ). For the light-dependent reactions, the interactive effect between light spectra and PPFD found for CO 2 assimilation and quantum yield was also observed for J ( Figure 8A ). At low PPFD (200 μmol⋅m –2 ⋅s –1 ), green light resulted in the lowest J and red light in the highest J among single waveband spectra. But at a PPFD of 1,000 μmol⋅m –2 ⋅s –1 , red and green light resulted in the highest J and blue light in the lowest J ( Figure 8A ), similar to the differences in A g .
There was no clear evidence of Rubisco limitations to photosynthesis at a PPFD of 200 μmol⋅m –2 ⋅s –1 , so the rate of the light-dependent reactions likely limited photosynthesis. This is corroborated by the strong correlation between A g and J at a PPFD of 200 μmol⋅m –2 ⋅s –1 . Although Rubisco limitations to photosynthesis were observed at a PPFD of 1,000 μmol⋅m –2 ⋅s –1 , there were no meaningful differences in V c,max in response to light spectrum, in contrast to J ( Figure 8 ).
When PPFD increased 5×, from 200 to 1,000 μmol⋅m –2 ⋅s –1 , there was only a 1.7 to 2.4× increase in J , indicating a lower QY(J) inc at higher PPFD . This matches the lower QY inc and the asymptotic increase in A n in response to increasing PPFD ( Figure 3 ). The relative increase of J under green light (143%) was greater than that under both blue and red light (73 and 75%, respectively) as PPFD increased. This similarly can be attributed to a more uniform energy distribution of green light among reaction centers throughout a leaf and weaker upregulation of non-photochemical quenching with increasing green light intensity ( Sun et al., 1998 ; Nishio, 2000 ; Evans and Vogelmann, 2003 ), as discussed before.
There was a strong correlation between J and A g under the nine light spectra at both PPFD levels ( Figure 9A ). QY abs and QY(J) abs are similarly strongly correlated ( Figure 9B ). Unlike J , V c,max was largely unaffected by light spectra ( Figure 8B ) and was not correlated with A g (data not shown). There was, however, a strong correlation between J and V c,max at a PPFD of 1,000 μmol⋅m –2 ⋅s –1 ( R 2 = 0.82, Supplementary Figure 3 ), suggesting that J and V c,max are co-regulated. Similarly, Wullschleger (1993) noted a strong linear relationship between J and V c,max across 109 C 3 species. The ratio between J and V c,max in our study (1.5–2.0) similar to the ratio found by Wullschleger (1993) . These results suggest that the interactive effect of light spectra and PPFD resulted from effects on J , which is associated with light energy harvesting by reaction centers, rather than from V c,max .
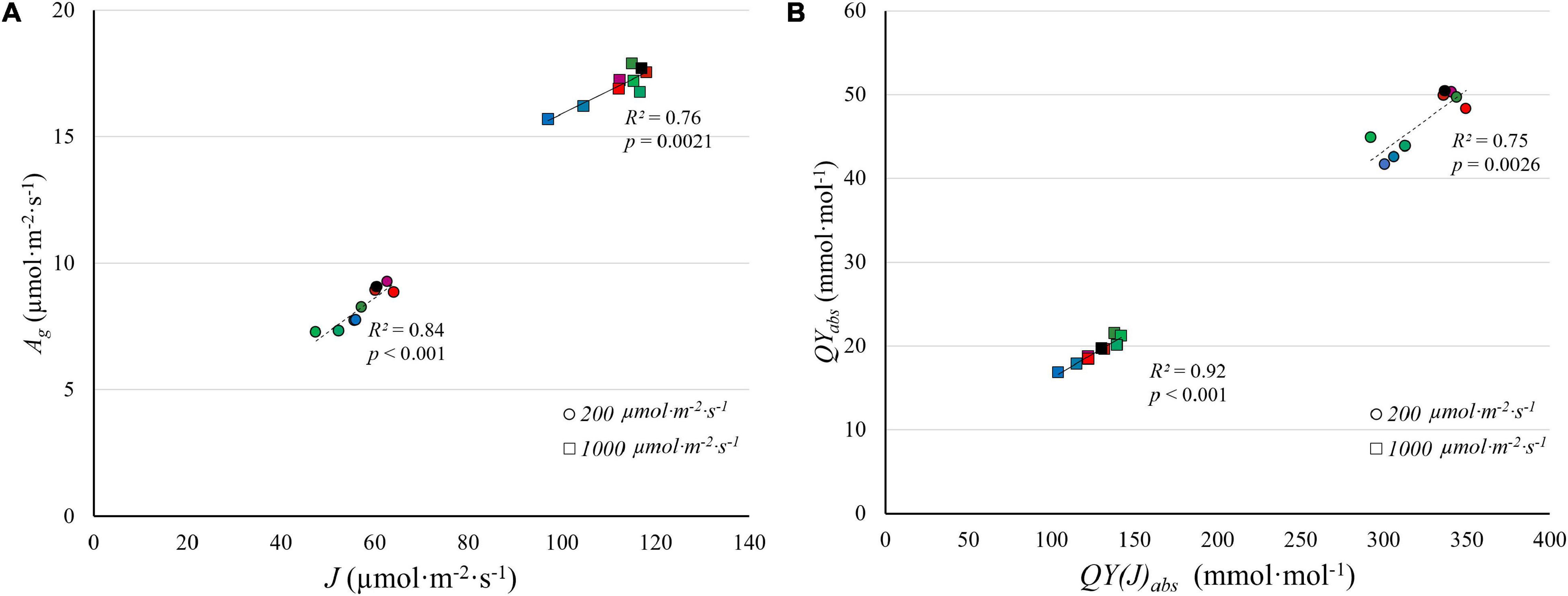
Figure 9. The correlation between gross CO 2 assimilation rate ( A g ) estimated from light response curves and electron transport rate ( J ) estimated from A/C i curves (A) , and between the quantum yield of CO 2 assimilation ( QY abs ) and the quantum yield of electron transport on an absorbed light basis [ QY(J) abs ] (B) , under low PPFD (200 μmol m –2 s –1 ) and high PPFD (1,000 μmol m –2 s –1 ) under nine light spectra averaged over nine “Green Towers” lettuce plants. The color scheme representing the nine spectra is the same as Figure 8 .
No Interactive Effects Among Blue, Green, and Red Light
The Emerson enhancement effect describes a synergistic effect between lights of different wavebands (red and far-red) on photosynthesis ( Emerson, 1957 ). McCree (1971) attempted to account for interactions between light with different spectra when developing photosynthetic action spectra and applied low intensity monochromatic lights from 350 to 725 nm with white background light to plants. His results showed no interactive effect between those monochromatic lights and white light ( McCree, 1971 ). We tested different ratios of blue, green, and red light and different PPFD s, and similarly did not find any synergistic or antagonistic effect of different wavebands on any physiological parameters measured or calculated.
Importance of Interactions Between PPFD and Light Quality and Its Applications
The interactive effect between PPFD and light quality demonstrates a remarkable adaptation of plants to different light intensities. By not absorbing green light strongly, plants open up a “green window,” as Terashima et al. (2009) called it, to excite chloroplasts deeper into leaves, and thus facilitating CO 2 assimilation throughout the leaf. While red light resulted in relatively high QY inc , QY abs and A n at both high and low PPFD ( Figures 3 , 6 ), it is still mainly absorbed in the upper part of leaves ( Sun et al., 1998 ; Brodersen and Vogelmann, 2010 ). Green light can penetrate deeper into leaves ( Brodersen and Vogelmann, 2010 ) and help plants drive efficient CO 2 assimilation at high PPFD ( Figures 3 , 5 ).
Many early photosynthesis studies investigated the absorptance and action spectrum of photosynthesis of green algae, e.g., Haxo and Blinks (1950) or chlorophyll or chloroplasts extracts, e.g., Chen (1952) . Extrapolating light absorptance of green algae and suspension of chlorophyll or chloroplast to whole leaves from can lead to an underestimation of absorptance of green light by whole leaves and the belief that green light has little photosynthetic activity ( Moss and Loomis, 1952 ; Smith et al., 2017 ). Photosynthetic action spectra developed on whole leaves of higher plants, however, have long shown that green light effectively contributes to CO 2 assimilation, although with lower QY inc than red light ( Hoover, 1937 ; McCree, 1971 ; Inada, 1976 ; Evans, 1987 ). The importance of green light for photosynthesis was clearly established in more recent studies, emphasizing its role in more uniformly exciting all chloroplasts, which especially important under high PPFD ( Sun et al., 1998 ; Nishio, 2000 ; Terashima et al., 2009 ; Hogewoning et al., 2012 ; Smith et al., 2017 ). The idea that red and blue light are more efficient at driving photosynthesis, unfortunately, still lingers, e.g., Singh et al. (2015) .
Light-emitting diodes (LEDs) have received wide attention in recent years for use in controlled environment agriculture, as they now have superior efficacy over traditional lighting technologies ( Pattison et al., 2018 ). LEDs can have a narrow spectrum and great controllability. This provides unprecedented opportunities to fine tune light spectra and PPFD to manipulate crop growth and development. Blue and red LEDs have higher efficacy than white and green LEDs ( Kusuma et al., 2020 ). By coincidence, McCree’s action spectrum ( Figure 1 ; McCree, 1971 ) also has peaks in the red and blue region, although the peak in the blue region is substantially lower than the one in the red region. Therefore, red and blue LEDs are sometimes considered optimal for driving photosynthesis. This claim holds true only under low PPFD . Green light plays an important role in photosynthesis, as it helps plants to adapt to different light intensities. The wavelength-dependent absorptance of chlorophylls channels green light deeper into leaves, resulting in more uniform light absorption throughout leaves and providing excitation energy to cells further from the adaxial surface. Under high PPFD , this can increase leaf photosynthesis. Plant evolved under sunlight for hundreds of millions of years, and it seems likely that the relatively low absorptance of green light contributes to the overall photosynthetic efficiency of plants ( Nishio, 2000 ).
There was an interactive effect of light spectrum and PPFD on leaf photosynthesis. Under low PPFD , QY inc was lowest under green and highest under red light. The low QY inc under green light at low PPFD was due to low absorptance. In contrast, at high PPFD , green and red light achieved similar QY inc , higher than that of blue light. The strong absorption of blue light by chlorophyll creates a large light gradient from the top to the bottom of leaves. The large amount of excitation energy near the adaxial side of a leaf results in upregulation of nonphotochemical quenching, while chloroplasts near the bottom of a leaf receive little excitation energy under blue light. The more uniform distribution of green light absorption within leaves reduces the need for nonphotochemical quenching near the top of the leaf, while providing more excitation energy to cells near the bottom of the leaf. We also found that the interactive effect of light spectrum and PPFD on photosynthesis was a result of the light-dependent reactions; gross assimilation and J were strongly correlated. We detected no synergistic or antagonistic interactions between blue, green, and red light.
Data Availability Statement
The raw data supporting the conclusions of this article will be made available by the authors, without undue reservation.
Author Contributions
JL and MI designed the experiment, discussed the data, and revised the manuscript. JL performed the experiment, analyzed data, and prepared the first draft. Both authors contributed to the article and approved the submitted version.
This study was funded by the USDA-NIFA-SCRI award number 2018-51181-28365, project Lighting Approaches to Maximize Profits.
Conflict of Interest
The authors declare that the research was conducted in the absence of any commercial or financial relationships that could be construed as a potential conflict of interest.
Supplementary Material
The Supplementary Material for this article can be found online at: https://www.frontiersin.org/articles/10.3389/fpls.2021.619987/full#supplementary-material
Supplementary Figure 1 | (Related to Figure 6 ) Quantum yield of CO 2 assimilation of “Green Towers” lettuce as a function of incident ( QY inc ) (A,C,E,G) and absorbed PPFD ( QY abs ) (B,D,F,H) under nine light spectra (see Table 1 ). Error bars represent standard deviation ( n = 9).
Supplementary Figure 2 | (Related to Figure 7 ) Differential quantum yield of CO 2 assimilation ( differential QY ) of “Green Towers” lettuce under nine light spectra as a function of the PPFD . Inserts show differential QY at PPFD s of 1,000–1,300 μmol⋅m –2 s –1 s to better show differences at high PPFD (note the different y -axis scale). The composition of the nine light spectra is shown in Table 1 . The light spectra in the graphs are (A) 100B, 100G and 100R; (B) 100B, 80B20G, 20B80G and 100G; (C) 100G, 80G20R, 20G80R and 100R; and (D) 20B80R, 16B20G64R and 100G.
Supplementary Figure 3 | (Related to Figure 6 ) The correlation between electron transport ( J ) and maximum Rubisco carboxylation rate ( V c,max ) of “Green Towers” lettuce estimated from A/C i curves under PPFD (1000 μmol m –2 s –1 ) under nine light spectra ( p < 0.001).
Supplementary Figure 4 | (Related to Figure 6 ) The comparison between QY inc before (A) and after (B) correcting for light-suppression of respiration under blue, green, and red LED light. Note that the initial increase in QY inc became more pronounced after correction of light suppressed respiration.
Supplementary Figure 5 | The comparison between QY abs before (A) and after (B) correcting for alternative electron sinks under blue, green, and red LED light. Assuming a simplified electron sink that diverts energy of 15 μmol m –2 s –1 of absorbed photons (an arbitrary value used for illustrative purposes only) away from the Calvin cycle under all PPFD s, the corrected QY abs was calculated based on remaining photons available to support Calvin cycle processes (B) . Note that the pattern of QY inc after correcting of alternative electron sink (B) is similar to quantum yield of PSII measured by chlorophyll fluorescence by Weaver and van Iersel (2019) .
Supplementary Table 1 | Dark respiration rate (R d ), maximum quantum yield of CO 2 assimilation (QY m,inc ) and maximum gross assimilation rate (A g,max ) of “Green towers” lettuce derived from the light response curves for nine different spectra using Eq. 1. The light response curves are shown in Figure 3 . *See light composition of nine lights presented here in Table 1 .
Abbreviations
PPFD , photosynthetic photon flux density; RuBP, ribulose 1,5-bisphosphate; Rubisco, ribulose-1,5-bisphosphate carboxylase/oxygenase; VPD, vapor pressure deficit; FWHM, full width at half maximum; A n , net CO 2 assimilation rate; R d , dark respiration rate; QY m,inc , maximum quantum yield of CO 2 assimilation; A g,max , light-saturated gross assimilation rate; QY m,abs , maximum quantum yield of CO 2 assimilation on absorbed light base; QY inc , quantum yield of CO 2 assimilation based on incident PPFD ; A g , gross CO 2 assimilation rate; QY abs , quantum yield of CO 2 assimilation on absorbed light base; QY , quantum yield of CO 2 assimilation; A/Ci curve, assimilation – internal leaf CO 2 response curve; RACiR, rapid A/C i response technique; V c,max , maximum rate of Rubisco carboxylation; J , rate of electron transport; CA1P, 2-carboxy- D -arabinitol-1-phosphate; NPQ, non-photochemical quenching.
Atkin, O. K., Westbeek, M., Cambridge, M. L., Lambers, H., and Pons, T. L. (1997). Leaf respiration in light and darkness (A comparison of slow- and fast-growing Poa species). Plant Physiol. 113, 961–965. doi: 10.1104/pp.113.3.961
PubMed Abstract | CrossRef Full Text | Google Scholar
Badger, M. R., von Caemmerer, S., Ruuska, S., and Nakano, H. (2000). Electron flow to oxygen in higher plants and algae: rates and control of direct photoreduction (Mehler reaction) and rubisco oxygenase. Philos. Trans. R. Soc. Lond. B Biol. Sci. 355, 1433–1446. doi: 10.1098/rstb.2000.0704
Balegh, S. E., and Biddulph, O. (1970). The photosynthetic action spectrum of the bean plant. Plant Physiol. 46, 1–5. doi: 10.1104/pp.46.1.1
Brodersen, C. R., and Vogelmann, T. C. (2010). Do changes in light direction affect absorption profiles in leaves? Funct. Plant Biol. 37, 403–412. doi: 10.1071/fp09262
CrossRef Full Text | Google Scholar
Brooks, A., and Farquhar, G. D. (1985). Effect of temperature on the CO2/O2 specificity of ribulose-1,5-bisphosphate carboxylase/oxygenase and the rate of respiration in the light. Planta 165, 397–406. doi: 10.1007/BF00392238
Campbell, W. J., and Ogren, W. L. (1992). Light activation of rubisco by rubisco activase and thylakoid membranes. Plant Cell Physiol. 33, 751–756. doi: 10.1093/oxfordjournals.pcp.a078314
Chen, S. L. (1952). The action spectrum for the photochemical evolution of oxygen by isolated chloroplasts. Plant Physiol. 27, 35–48. doi: 10.1104/pp.27.1.35
Craver, J. K., Nemali, K. S., and Lopez, R. G. (2020). Acclimation of growth and photosynthesis in Petunia seedlings exposed to high-intensity blue radiation. J. Am. Soc. Hortic. Sci. 145, 152–161. doi: 10.21273/jashs04799-19
Croce, R., Müller, M. G., Bassi, R., and Holzwarth, A. R. (2001). Carotenoid-to-chlorophyll energy transfer in recombinant major light-harvesting complex (LHCII) of higher plants. I. Femtosecond transient absorption measurements. Biophys. J. 80, 901–915. doi: 10.1016/S0006-3495(01)76069-9
de Weerd, F. L., Dekker, J. P., and van Grondelle, R. (2003a). Dynamics of β-carotene-to-chlorophyll singlet energy transfer in the core of photosystem II. J. Phys. Chem. B 107, 6214–6220. doi: 10.1021/jp027737q
de Weerd, F. L., Kennis, J. T., Dekker, J. P., and van Grondelle, R. (2003b). β-Carotene to chlorophyll singlet energy transfer in the photosystem I core of Synechococcus elongatus proceeds via the β-carotene S2 and S1 states. J. Phys. Chem. B 107, 5995–6002. doi: 10.1021/jp027758k
Emerson, R. (1957). Dependence of yield of photosynthesis in long-wave red on wavelength and intensity of supplementary light. Science 125, 746–746. doi: 10.1126/science.125.3251.746
Evans, J. (1987). The dependence of quantum yield on wavelength and growth irradiance. Funct. Plant Biol. 14, 69–79. doi: 10.1071/PP9870069
Evans, J., and Vogelmann, T. C. (2003). Profiles of 14C fixation through spinach leaves in relation to light absorption and photosynthetic capacity. Plant Cell Environ. 26, 547–560. doi: 10.1046/j.1365-3040.2003.00985.x
Haxo, F. T., and Blinks, L. (1950). Photosynthetic action spectra of marine algae. J. Gen. Physiol. 33, 389–422. doi: 10.1085/jgp.33.4.389
Hogewoning, S. W., Wientjes, E., Douwstra, P., Trouwborst, G., Van Ieperen, W., Croce, R., et al. (2012). Photosynthetic quantum yield dynamics: from photosystems to leaves. Plant Cell 24, 1921–1935. doi: 10.1105/tpc.112.097972
Hoover, W. H. (1937). The dependence of carbon dioxide assimilation in a higher plant on wave length of radiation. Smithson. Misc. Collect. 95, 1–13.
Google Scholar
Inada, K. (1976). Action spectra for photosynthesis in higher plants. Plant Cell Physiol. 17, 355–365. doi: 10.1093/oxfordjournals.pcp.a075288
Krall, J. P., and Edwards, G. E. (1992). Relationship between photosystem II activity and CO2 fixation in leaves. Physiol. Plant. 86, 180–187. doi: 10.1111/j.1399-3054.1992.tb01328.x
Kusuma, P., Pattison, P. M., and Bugbee, B. (2020). From physics to fixtures to food: current and potential LED efficacy. Hortic. Res. 7:56. doi: 10.1038/s41438-020-0283-7
McCree, K. J. (1971). The action spectrum, absorptance and quantum yield of photosynthesis in crop plants. Agric. Meteorol. 9, 191–216. doi: 10.1016/0002-1571(71)90022-7
McCree, K. J. (1972). Test of current definitions of photosynthetically active radiation against leaf photosynthesis data. Agric. Meteorol. 10, 443–453. doi: 10.1016/0002-1571(72)90045-3
Moss, R. A., and Loomis, W. E. (1952). Absorption spectra of leaves. I. the visible spectrum. Plant Physiol. 27, 370–391. doi: 10.1104/pp.27.2.370
Müller, J., Wernecke, P., and Diepenbrock, W. (2005). LEAFC3-N: a nitrogen-sensitive extension of the CO 2 and H 2 O gas exchange model LEAFC3 parameterised and tested for winter wheat (Triticum aestivum L.). Ecol. Modell. 183, 183–210. doi: 10.1016/j.ecolmodel.2004.07.025
Nishio, J. (2000). Why are higher plants green? Evolution of the higher plant photosynthetic pigment complement. Plant Cell Environ. 23, 539–548. doi: 10.1046/j.1365-3040.2000.00563.x
Nunes-Nesi, A., Fernie, A. R., and Stitt, M. (2010). Metabolic and signaling aspects underpinning the regulation of plant carbon nitrogen interactions. Mol. plant 3, 973–996.
Parry, M. A., Keys, A. J., Madgwick, P. J., Carmo-Silva, A. E., and Andralojc, P. J. (2008). Rubisco regulation: a role for inhibitors. J. Exp. Bot. 59, 1569–1580. doi: 10.1093/jxb/ern084
Pattison, P., Lee, K., Stober, K., and Yamada, M. (2018). Energy Savings Potential of SSL in Horticultural Applications. Washington, DC: U.S. Department of Energy, Office of Scientific and Technical Information.
Sharkey, T. D., Bernacchi, C. J., Farquhar, G. D., and Singsaas, E. L. (2007). Fitting photosynthetic carbon dioxide response curves for C3 leaves. Plant Cell Environ. 30, 1035–1040. doi: 10.1111/j.1365-3040.2007.01710.x
Singh, D., Basu, C., Meinhardt-Wollweber, M., and Roth, B. (2015). LEDs for energy efficient greenhouse lighting. Renew. Sustain. Energy Rev. 49, 139–147.
Skillman, J. B. (2008). Quantum yield variation across the three pathways of photosynthesis: not yet out of the dark. J. Exp. Bot. 59, 1647–1661. doi: 10.1093/jxb/ern029
Smith, H. L., McAusland, L., and Murchie, E. H. (2017). Don’t ignore the green light: exploring diverse roles in plant processes. J. Exp. Bot. 68, 2099–2110. doi: 10.1093/jxb/erx098
Stinziano, J. R., Morgan, P. B., Lynch, D. J., Saathoff, A. J., McDermitt, D. K., and Hanson, D. T. (2017). The rapid A–Ci response: photosynthesis in the phenomic era. Plant Cell Environ. 40, 1256–1262. doi: 10.1111/pce.12911
Sun, J., Nishio, J. N., and Vogelmann, T. C. (1998). Green light drives CO2 fixation deep within leaves. Plant Cell Physiol. 39, 1020–1026. doi: 10.1093/oxfordjournals.pcp.a029298
Takahashi, H., Kopriva, S., Giordano, M., Saito, K., and Hell, R. (2011). Sulfur assimilation in photosynthetic organisms: molecular functions and regulations of transporters and assimilatory enzymes. Annu. Rev. Plant Biol. 62, 157–184. doi: 10.1146/annurev-arplant-042110-103921
Terashima, I., Fujita, T., Inoue, T., Chow, W. S., and Oguchi, R. (2009). Green light drives leaf photosynthesis more efficiently than red light in strong white light: revisiting the enigmatic question of why leaves are green. Plant cell physiol. 50, 684–697. doi: 10.1093/pcp/pcp034
Vogelmann, T., and Han, T. (2000). Measurement of gradients of absorbed light in spinach leaves from chlorophyll fluorescence profiles. Plant Cell Environ. 23, 1303–1311. doi: 10.1046/j.1365-3040.2000.00649.x
Vogelmann, T. C., and Evans, J. (2002). Profiles of light absorption and chlorophyll within spinach leaves from chlorophyll fluorescence. Plant Cell Environ. 25, 1313–1323. doi: 10.1046/j.1365-3040.2002.00910.x
Vogelmann, T. C., and Gorton, H. L. (2014). “Leaf: light capture in the photosynthetic organ,” in The Structural Basis of Biological Energy Generation , ed. M. F. Hohmann-Marriott, (Dordrecht: Springer Netherlands), 363–377.
Weaver, G., and van Iersel, M. W. (2019). Photochemical characterization of greenhouse-grown Lettuce ( Lactuca sativa L. ‘Green Towers’) with applications for supplemental lighting control. HortScience 54, 317–322. doi: 10.21273/hortsci13553-18
Wientjes, E., van Stokkum, I. H., van Amerongen, H., and Croce, R. (2011). The role of the individual Lhcas in photosystem I excitation energy trapping. Biophys. J. 101, 745–754. doi: 10.1016/j.bpj.2011.06.045
Wullschleger, S. D. (1993). Biochemical limitations to carbon assimilation in C3 Plants—a retrospective analysis of the A/Ci curves from 109 species. J. Exp. Bot. 44, 907–920. doi: 10.1093/jxb/44.5.907
Zhang, N., and Portis, A. R. (1999). Mechanism of light regulation of rubisco: a specific role for the larger rubisco activase isoform involving reductive activation by thioredoxin-f. Proc. Natl. Acad. Sci. U.S.A. 96, 9438–9443. doi: 10.1073/pnas.96.16.9438
Zhen, S., Haidekker, M., and van Iersel, M. W. (2019). Far−red light enhances photochemical efficiency in a wavelength−dependent manner. Physiol. Plant. 167, 21–33. doi: 10.1111/ppl.12834
Zhen, S., and van Iersel, M. W. (2017). Photochemical acclimation of three contrasting species to different light levels: implications for optimizing supplemental lighting. J. Am. Soc. Hortic. Sci. 142, 346–354. doi: 10.21273/jashs04188-17
Keywords : photosynthesis, quantum yield of CO 2 assimilation, light spectrum, photosynthetic photon flux density, electron transport, V c, max , light intensity, light quality
Citation: Liu J and van Iersel MW (2021) Photosynthetic Physiology of Blue, Green, and Red Light: Light Intensity Effects and Underlying Mechanisms. Front. Plant Sci. 12:619987. doi: 10.3389/fpls.2021.619987
Received: 21 October 2020; Accepted: 11 February 2021; Published: 05 March 2021.
Reviewed by:
Copyright © 2021 Liu and van Iersel. This is an open-access article distributed under the terms of the Creative Commons Attribution License (CC BY) . The use, distribution or reproduction in other forums is permitted, provided the original author(s) and the copyright owner(s) are credited and that the original publication in this journal is cited, in accordance with accepted academic practice. No use, distribution or reproduction is permitted which does not comply with these terms.
*Correspondence: Jun Liu, [email protected]
Disclaimer: All claims expressed in this article are solely those of the authors and do not necessarily represent those of their affiliated organizations, or those of the publisher, the editors and the reviewers. Any product that may be evaluated in this article or claim that may be made by its manufacturer is not guaranteed or endorsed by the publisher.
Click through the PLOS taxonomy to find articles in your field.
For more information about PLOS Subject Areas, click here .
Loading metrics
Open Access
Peer-reviewed
Research Article
The effect of light quality on plant physiology, photosynthetic, and stress response in Arabidopsis thaliana leaves
Roles Conceptualization, Data curation, Formal analysis, Methodology, Visualization, Writing – original draft
* E-mail: [email protected] (ML); [email protected] (NY)
Affiliation Department of Bioresource Engineering, McGill University–Macdonald Campus, Sainte-Anne-de-Bellevue, Quebec, Canada

Roles Methodology
Affiliation Department of Plant Science, McGill University–Macdonald Campus, Sainte-Anne-de-Bellevue, Quebec, Canada
Roles Writing – review & editing
Roles Resources
Roles Conceptualization, Funding acquisition, Investigation, Project administration, Resources, Supervision, Writing – original draft
- Nafiseh Yavari,
- Rajiv Tripathi,
- Bo-Sen Wu,
- Sarah MacPherson,
- Jaswinder Singh,
- Mark Lefsrud
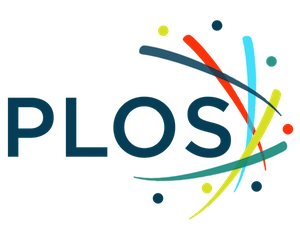
- Published: March 4, 2021
- https://doi.org/10.1371/journal.pone.0247380
- Reader Comments
The impacts of wavelengths in 500–600 nm on plant response and their underlying mechanisms remain elusive and required further investigation. Here, we investigated the effect of light quality on leaf area growth, biomass, pigments content, and net photosynthetic rate (Pn) across three Arabidopsis thaliana accessions, along with changes in transcription, photosynthates content, and antioxidative enzyme activity. Eleven-leaves plants were treated with BL; 450 nm, AL; 595 nm, RL; 650 nm, and FL; 400–700 nm as control. RL significantly increased leaf area growth, biomass, and promoted Pn. BL increased leaf area growth, carotenoid and anthocyanin content. AL significantly reduced leaf area growth and biomass, while Pn remained unaffected. Petiole elongation was further observed across accessions under AL. To explore the underlying mechanisms under AL, expression of key marker genes involved in light-responsive photosynthetic reaction, enzymatic activity of antioxidants, and content of photosynthates were monitored in Col-0 under AL, RL (as contrast), and FL (as control). AL induced transcription of GSH2 and PSBA , while downregulated NPQ1 and FNR2 . Photosynthates, including proteins and starches, showed lower content under AL. SOD and APX showed enhanced enzymatic activity under AL. These results provide insight into physiological and photosynthetic responses to light quality, in addition to identifying putative protective-mechanisms that may be induced to cope with lighting-stress in order to enhance plant stress tolerance.
Citation: Yavari N, Tripathi R, Wu B-S, MacPherson S, Singh J, Lefsrud M (2021) The effect of light quality on plant physiology, photosynthetic, and stress response in Arabidopsis thaliana leaves. PLoS ONE 16(3): e0247380. https://doi.org/10.1371/journal.pone.0247380
Editor: Keqiang Wu, National Taiwan University, TAIWAN
Received: August 31, 2020; Accepted: December 13, 2020; Published: March 4, 2021
Copyright: © 2021 Yavari et al. This is an open access article distributed under the terms of the Creative Commons Attribution License , which permits unrestricted use, distribution, and reproduction in any medium, provided the original author and source are credited.
Data Availability: All relevant data are within the manuscript and its Supporting Information files.
Funding: This work was supported by the “Natural Sciences and Engineering Research Council of Canada (NSERC)”. The specific grant number is RGPIN 355743-13, CRDPJ418919-11. It is “all” the funding and/or financial sources of support (whether external and/or internal to our organization) that were received during this study. And there was no additional external and/or internal funding received for this study. ML is the author, who received this award. The funders had no role in study design, data collection and analysis, decision to publish, or preparation of the manuscript.
Competing interests: The authors declare that the research was conducted in the absence of any commercial or financial relationships that could be construed as a potential conflict of interest.
Introduction
Among various environmental factors, light is one of the most important variables affecting photosynthesis as well as plant growth and development [ 1 ]. Plants require light not only as an energy source but also as a clue to adjust their development to environmental conditions. During photosynthesis, absorbed energy is transferred to the photosynthetic apparatus, which is comprised of Photosystem I (PSI), Photosystem II (PSII), electron transport carriers (cytochrome b6f (cytb6f), plastoquinone (PQ), plastocyanin (PC)), and ATP synthase. The light-responsive photosynthetic process is driven by the released electrons through the water-splitting reaction on the PSII side, followed by NADP + reduction to NADPH, and proton flow into the lumen in order to generate ATP. Generated NADPH and ATP serve as an energy source for the carbon fixation process [ 2 ].
Both quality and quantity of incident light can have drastic impacts on photosynthetic activity and photosystem adaption to changing light quality [ 3 , 4 ]. Earlier studies on photosynthetic activity reported that photosynthesis is a wavelength-dependent response, in which amber light (AL; 595 nm) induces higher photosynthetic rates than blue light (BL; 450 nm) or red light (RL; 650 nm) [ 3 , 5 , 6 ]. These studies have become the foundation for our plant lighting research as light emitting diodes (LEDs) are proven to be an optimal and effective tool to study the effect of wavelength on plant physiological and biochemical responses [ 7 – 10 ]. Prior research has demonstrated that the wavelength range from 430–500 nm is effective at simulating pigmentation, metabolism of secondary metabolites, photosynthetic function, and development of chloroplasts [ 11 – 14 ]. The wavelength range of 640–670 nm was found effective in promoting photosynthetic activity, plant biomass and leaf area growth [ 3 , 15 ] while playing critically important roles in the development of photosynthetic apparatus, net photosynthetic rate (Pn) and primary metabolism [ 12 , 16 ]. Growing research on the wavelength range 500–600 nm have highlighted its important physiological and morphological impact on growth, chlorophyll content, and photosynthetic function [ 8 , 17 – 19 ]. However, conflicting results on the impact of AL were reported [ 3 , 20 ]. Although AL results in high photosynthetic activity, poor plant growth responses such as elongation and growth suppression have been reported [ 20 , 21 ], and this underlying mechanism remains unknown. In addition to this, AL is weakly absorbed by the photosynthetic pigments [ 22 ]. At the current state, further investigation of AL impact is required to better understand the photoactivity of the photosystems.
Recent studies reported that light quality and quantity can have drastic impacts on imbalanced excitation of either PSII or PSI, resulting in energy imbalance between photosystems and triggering stoichiometric adjustments of photosynthetic complexes [ 23 , 24 ]. This imbalance between the two photosystems can result in generation of harmful reactive intermediates, mainly reactive oxygen species (ROS) [ 25 , 26 ]. Generation of ROS can result in oxidative damage to the chloroplasts, leading to photosystem photo-inhibition that strongly limits plant growth [ 27 ]. To maintain steady state photosynthetic efficiency and prevent ROS accumulation, plants activate the buffering mechanisms, including cyclic photosynthetic electron flow (CEF) and non-photochemical quenching (NPQ) [ 28 , 29 ]. To scavenge ROS, plants further stimulate antioxidative mechanisms via enhanced activity of associated enzymes such as glutathione synthetase (GSH), ascorbate peroxidase (APX), and superoxide dismutase (SOD) [ 30 ]. These studies and their findings allow us to understand the impact of light within photosystems; however, the wavelength that can induce such stress responses and their physiological consequence on plants remain poorly studied.
Therefore, to better understand the effect of light quality on plant growth and photosynthetic performance, we studied three narrow-wavelength LEDs of blue light (BL; 450 nm), amber light (AL; 595 nm), and red light (RL; 650 nm), and compared them with fluorescent light (FL; 400–700) as the control. We chose light quality of BL and RL as leaf pigments have absorption peaks at these wavelengths [ 31 ]. AL was chosen due to the conflicting results between high photosynthetic activity and poor plant growth responses [ 3 , 5 ]. Furthermore, to assess whether light quality-induced changes in plant growth and photosynthesis are mediated by the genotype, we investigated the light quality response in three A . thaliana accessions Col-0, Est-1, and C24. These accessions show different geographical distributions and hence are adopted to different environments [ 32 – 34 ]. Congruently, they show a high degree of divergence in their photosynthetic response to the light environment [ 35 , 36 ]. Two experiments were designed to assess the impact of light quality on the plant. First, we investigated the physiological and photosynthetic response of A . thaliana to BL, AL, and RL lights compared it to FL by measuring leaf area growth, biomass content, Pn, and pigments content. Second, we tested whether changes in plant response to light quality is genotype specific by conducting the experiments across three A . thaliana accessions. Third, we investigated the potential induction of stress responses under AL by testing whether there are light quality-specific changes in the expression of marker genes involved in light-responsive photosynthetic process and enzymatic activity of antioxidants, as well as photosynthates content. Our findings expand the current understanding on physiological and photosynthetic responses of plants to light quality, in addition to identifying putative protective-mechanisms that may be induced to cope with lighting-stress in order to enhance plant stress tolerance.
Materials and methods
Plant materials and growth condition.
Seeds of A . thaliana accessions Col-0, Est-1, and C24 were obtained from the Arabidopsis Biological Resource Center (ABRC; Columbus, OH, US). Seeds were placed in rockwool cubes (Grodan A/S, DK-2640, Hedehusene, Denmark) and incubated at 4°C for 2 days. White broad-spectrum light (FL; 4200 K, F72T8CW, Osram Sylvania, MA, US) were used as light sources for seed germination. Seedlings were hydroponically grown under FL for 21 days with the environmental condition of 24 h photoperiod, 23°C, 50% relative humidity, and ambient CO 2 in a growth chamber (TC30, Conviron, Winnipeg, MB, Canada). Seed density was adjusted to limit treated plants from shadowing each other. FL was placed over the plant-growing surface area (49 cm × 95 cm) at a low photosynthetic photon flux density (PPFD) of 69 to 71 μmol·m -2 ·sec -1 . PPFD was measured at the conjunction of a grid (square area 3 cm 2 ) placed over the growing area. After 21 days, plants formed rosettes with nine (C24) and eleven (Col-0 and Est-1) leaves. To reach the same growth stage as Col-0 and Est-1 plants, C24 plants were allowed to grow for 23 days [ 37 ]. Fresh half-strength Hoagland nutrient solution [ 38 ] was provided every other day.
Light treatment
After day 21 (Col-0 and Est-1) or 23 (C24), plants were transferred to their respective light treatment for 5 days, each with the same environmental conditions: 24 h photoperiod, 23°C, 50% relative humidity, and ambient CO 2 . 21-day old plants were randomly divided into four experimental groups and received treatments using light emitting diodes (LED) (VanqLED, Shenzhen, China) of BL (peak wavelength: 450 nm), AL (peak wavelength: 595 nm), and RL (peak wavelength: 650 nm). The fourth group was treated with FL (400–700 nm), as the control. The light spectra and PPFD were monitored daily by using a PS-300 spectroradiometer (Apogee, Logan, UT, US). PPFD was maintained at 69 to 71 μmol·m -2 ·sec -1 throughout the whole plant growth period. Fresh half-strength Hoagland nutrient solution [ 38 ] was provided every other day. Biological replicates were grown at different time points under the same environmental settings.
Physical and biochemical analyses
Leaf area growth determination..
Three plants per biological replicate were randomly selected for each measurement. Leaves from the selected plants were collected for the determination after treatment (5 days). Digital images of leaves were taken with a window size of 640 x 480 pixels and a camera-object distance of approximately 80 cm. The digital images were next used to determine leaf area growth using Image J software with default settings (Bethesda, MD, US), as described previously [ 39 ].
Biomass content determination.
Three plants per biological replicate were randomly selected for each determination. Leaf samples from the selected plants were collected for the dry mass determination before (0 h) and after treatment (5 days). Leaves were dried at 80°C for 2 days until a constant mass was achieved (less than < 5% mass difference over a 2 h period).
Pigment content determination.
Five plants per biological replicate were randomly selected for each assay. Leaf samples from the selected plants were collected for the determination after treatment (5 days). Methods and equations described by [ 40 – 42 ] were used to estimate the content of chlorophyll (Chl a and Chl b), carotenoids, and anthocyanin, respectively. Briefly, chlorophylls and carotenoids were extracted with 5 ml of 80% acetone at 4°C overnight, before centrifugation at 13,000 g for 5 min. Total anthocyanins were determined by extracting with 5 ml 80% methanol containing 1% HCl solvent at 4°C overnight, before centrifugation at 13,000 g for 5 min. The absorbance of the extraction solution was determined for Chl a (664 nm), Chl b (647 nm), carotenoids (440 nm), and anthocyanins (530 nm and 657 nm) using a UV–VIS spectrophotometer (UV-180, Shimadzu, Japan).
Net photosynthetic rate determination
Net photosynthetic rate was monitored before (0 h) and after treatment (5 days) using the LI-6400XT Portable Photosynthesis System (LI-COR Biosciences, Lincoln, NE, US) equipped with a 6400–17 Whole Plant Arabidopsis Chamber (LI-COR Biosciences). To reduce potential measurement errors, three plants were grouped as a single sample for determinations [ 43 ]. To avoid mismatch between the light quality used by the LI-6400XT Portable Photosynthetic System, and the LED lights used for the treatments [ 44 ], measurements were taken inside the controlled-chamber, in which whole plants (still embedded in rockwool) were placed and illuminated with LEDs. As a precaution, parafilm was placed on top of the rockwool cube to maintain moisture within the root zone while measurements were recorded. The environmental conditions of the chamber were set as: 400 ppm CO 2 , 50% relative humidity, 23°C, and 400 μl min -1 flow rate. Each measurement was taken over 20 min, including 5 min in the dark and 10–15 min under a light treatment at 69–71 μmol·m -2 ·sec -1 . A stable Pn reading was reached 10 min after illumination. Leaf area growth was determined to normalize Pn per unit leaf area growth. Measurements for three replicates (three plants per replicate, three replicates per treatment) were performed.
Photosynthate content determination
Previous studies have reported that the diurnal cycle and developmental stage of plants, along with the stress response can affect the plant metabolism [ 45 , 46 ]. Thus, we performed a time course assessment of 0, 1, 3, 5, and 7 days to determine the content of leaf photosynthates (proteins, starches, and lipids). Five plants per biological replicate were randomly selected for each measurement. Leaf samples from selected plants were collected for the determination prior (0 h) and after light treatments (1, 3, 5, and 7 days). Samples were immediately frozen in liquid nitrogen and stored at -80 ∘ C, before they were used for determination. Protein : Total protein content was measured using the Pierce™ BCA Protein Assay Kit (Thermo Fisher Scientific, Rockford, IL, USA). As a standard, the absorbance of the bovine serum albumin was determined at UV/Vis: λ max 562 nm. Starch : A previously described method [ 47 ] was used to estimate total starch content. Lipids : Previously described methods [ 48 , 49 ] were used (with minor modifications) to estimate the total lipid content. Briefly, each sample was homogenized with (CHCl 3 /MeOH, 70:30 v/v), before centrifuged at 1000 rpm for 5 min. The collected supernatant was incubated for 30 min at 70°C in a boiling water bath. Next, (H 2 SO 4 : 1 ml) was added and heated for 20 min. Following 2 min cooling on ice, (H 3 PO 4 : 1.5 ml) was added and incubated for 10 min until a pink color developed.
Antioxidative enzyme activity estimation
Five plants per biological replicate were randomly selected for each measurement. Leaf samples from selected plants were collected for the determination after treatment (5 days). Samples were immediately frozen in liquid nitrogen and stored at -80 ∘ C, before they were used for determination. Methods described by [ 50 , 51 ] were used to monitor the activity of SOD and APX antioxidative enzymes, respectively. Enzymatic activity was measured for 5 min at room temperature. The protein content in the supernatant was determined by the Pierce™ BCA Protein Assay Kit. The activity of SOD and APX was expressed as unit min −1 mg −1 protein.
Gene expression analysis
Cdna synthesis..
Changes in transcription of the interested genes were analyzed in A . thaliana Col-0 treated for 24 h under AL, RL, and FL. Leaf samples from selected plants were collected for the determination prior to treatment (0 h) and after treatment (2 h, 4 h, and 24 h). Samples were immediately frozen in liquid nitrogen and stored at -80 ∘ C, before they were used for determination. Four biological replicates were examined. For each biological replicate, five A . thaliana plants were selected, and their leaves were pooled together to represent a biological replicate. Plants in each biological replicate were grown independently, and at different times. Total RNA was extracted from (100 mg) leaves using the Sigma Spectrum Plant Total RNA Kit (STRN50; Sigma, Seelze, Germany) according to the manufacturer’s protocol. A total of (2 μg) RNA per sample was treated with amplification grade DNase I (Invitrogen, Carlsbad, CA, USA) to remove any traces of genomic DNA contamination. RNA concentrations were measured before and after DNase I digestion with a NanoDrop ND-1000 UV-Vis spectrophotometer (NanoDrop Technologies, Wilmington, Delware, USA). The cDNA was synthesized using AffinityScript QPCR cDNA Synthesis Kit (Agilent, Tech., Santa Clara, USA).
Primer design.
Primers for genes of interest ( S1 Table ) were designed using IDT software ( https://www.idtdna.com/calc/analyzer ) with the following criteria: Tm of 58–60°C and PCR amplicon lengths of 70 to 120 bp, yielding primer sequences 20 to 25 nucleotides in length with G-C contents of 40% to 50%. Specificity of the resulting primer pair sequences was examined using Arabidopsis transcript database with TAIR BLAST ( http://www.arabidopsis.org/Blast/ ). Specificity of the primer amplicons was further confirmed by melting-curve analysis (30 amplification cycles by PCR and subsequent gel-electrophoretic analysis). Primer amplicons were resolved on (agarose gels, 2% w/v) run at 110 V in Tris-borate/EDTA buffer, along with a 1Kb + DNA-standard ladder (Invitrogen, Carlsbad, CA, USA).
Quantitative real time-PCR (qRT-PCR) analysis.
Real-time qRT-PCR was performed with a MX3000P qPCR System (Agilent, Tech., Santa Clara, CA, USA) using three biological and two technical replicates, as described previously [ 52 ]. Relative expression was conducted following the manufacturer’s recommendations with two reference genes gamma tonoplast intrinsic protein 2 ( TIP2 ; AT3g26520) and actin 2 ( ACT2 ; AT3g18780) and the Brilliant III SYBR Green QPCR master mix (Agilent, Tech., Santa Clara, CA, USA). Amplification was performed in a (20 μL) reaction mixture containing (160 nmol) for each primer, 1x Brilliant III SYBR Green QPCR master mix, (15μM) ROX reference dye, and (0.3 μL) of cDNA template. Amplification conditions were 95°C for 10 min (hot start), followed by 40 cycles at 94°C for 30 s, 60°C for 30 s, and 72°C for 30 s. Fluorescence readings were taken at 72°C, at the end of the elongation cycle.
Data analysis.
Ct values were calculated with CFX-Manager and MX-3000P software. Relative expression changes (delta-delta Ct) were calculated according to [ 53 ] using A . thaliana TIP2 (AT3g26520) and ACT2 (AT3g18780) as reference genes. To avoid multiple testing, the p-values were only considered for 0 h with 24 h (a total of 12 genes and two light conditions). A gene was considered differentially expressed if p < 0.05 and the fold change pattern at 24 h was consistent with those observed at 2 and 4 h.
Statistical analysis
Differences between light treatments were tested using the two-tailed Student’s t-test. A two-way ANOVA was used to assess the effects of accession and different light treatments on leaf area growth, biomass content, Pn value, and pigments content. We observed similar patterns using the non-parametric tests of Wilcoxon-Mann-Whitney and Kruskal-Wallis tests (data not shown).
Effect of light quality and natural genotype variation on leaf area growth, biomass content, net photosynthetic rate, and pigment content in A . thaliana
To assess the effect of light quality, 21-days-old plants (11 leave plants) of three A . thaliana accessions Col-0, Est-1, and C24 were randomly divided into groups and treated under narrow-spectrum light (BL, AL, and RL), along with FL as control (baseline), for 5 days at approximately 70 μmol m -2 sec -1 ( Fig 1A and 1B ). Summary of light quantity compositions emitted from FL and LEDs light sources are shown in Table 1 . After 5 days of narrow-spectrum light treatments, leaf area growth, leaf biomass (dry mass), net photosynthetic rate, and pigment contents were measured across three A . thaliana accessions and compared with the baseline FL treatment ( Fig 1C–1E and Table 2 ).
- PPT PowerPoint slide
- PNG larger image
- TIFF original image
(A) Light emission spectra of LED light sources and FL. (B) Eleven-leaves stage A . thaliana accessions Col-0, Est-1, and C24 were grown hydroponically and treated for 5 days under narrow-spectrum BL, AL, and RL lights, as well as FL as control. (C) Leaf area growth. (D) Leaf biomass (dry mass). (E) Net photosynthetic rate (Pn) measured at 69–71 μmol m -2 sec -1 . Data are expressed as mean values ± standard deviation (n = 3). Statistical analysis was performed against FL using a two-tailed Student’s t-test (n.s.: not statistically significant; *: P < 0.05; **: P < 0.01).
https://doi.org/10.1371/journal.pone.0247380.g001
https://doi.org/10.1371/journal.pone.0247380.t001
https://doi.org/10.1371/journal.pone.0247380.t002
Under RL, the leaf area growth was significantly increased across accessions ( P < 0.05; Fig 1C ). Under BL, leaf area growth was significantly increased in C24 and Col-0 ( P < 0.05; Fig 2C ), but the increase in the leaf area growth was not significant in Est-1. Under AL, leaf area growth showed a severe reduction in Col-0 and C24 ( P < 0.05; Fig 1C ), while Al showed no change in Est-1. Petioles were noticeably elongated under AL ( Fig 1B ).
https://doi.org/10.1371/journal.pone.0247380.g002
The leaf biomass significantly increased under RL across the three accessions ( P < 0.05; Fig 1D ). Under BL, the leaf biomass was significantly decreased in Est-1 and C24 but increased in Col-0 ( P < 0.01; Fig 1D ). Under AL, the leaf biomass was significantly lower in Col-0 and C24 ( P < 0.01), while it showed no change in Est-1 ( Fig 1D ).
As for the net photosynthetic rates (Pn), it significantly increased under RL across the accessions ( P < 0.05; Fig 1E ). In contrast, there was no significant difference in Pn under AL ( Fig 1E ). Under BL, Pn significantly increased in Col-0 and Est-1 ( P < 0.05; Fig 1E ) but remained unchanged in C24.
There was no significant difference in contents of chlorophyll a (Chl a) and chlorophyll b (Chl b) in Col-0 and C24 under the light quality of BL, AL, and RL ( Table 2 ). In contrast, Chl a content significantly increased in Est-1 under RL ( P < 0.05; Table 2 ). Across accessions, Chl a: b content significantly increased, remained unchanged, and decreased under RL, BL, and AL, respectively ( Table 2 ). Moreover, there was no significant difference in carotenoid and anthocyanin contents across the accessions under AL and RL. However, BL significantly stimulated carotenoids content in Est-1 and Col-0 ( P < 0.05; Table 2 ). Additionally, anthocyanins content significantly increased under BL in Est-1 and C24 ( P < 0.01; Table 2 ).
The two-way ANOVA analysis indicated significant effects of the light treatments for the determined parameters, except Chl b. Also, the interaction between light treatments and genotype was significant for leaf area growth and leaf biomass ( P < 0.01; Table 3 ).
https://doi.org/10.1371/journal.pone.0247380.t003
Changes in transcription of photosynthetic marker genes, content of photosynthates, and activity of antioxidant in A . thaliana Col-0 under AL and RL
The severe reduction in leaf area growth and biomass, along with unchanged levels of Pn in Col-0 and C24 under AL suggested that amber light has mismatched effects on photosynthetic activity and photomorphology. Further to this, although chlorophyll contents under AL were 10–20% lower than the FL, both light treatments triggered similar photosynthetic activity, which implies that amber light has unidentified mechanisms in the photosynthetic process. To identify the mechanisms that amber light triggers within plants, we next explored transcriptional changes in marker genes associated with the photosynthetic light reaction and photo-protective mechanisms, photosynthates content and antioxidant enzymatic activity in Col-0 under AL ( Fig 2 ). Among three accession, Accession Col-0 was chosen for the transcription analysis, as it is the most common A . thaliana accession in conducting biological analysis. In addition to AL and FL (as control), changes were investigated under RL, as RL-treated plants showed opposing changes in leaf physiological phenotypes compared to AL.
Gene expression analysis indicated a significant increase in transcription level of ATP synthase gamma chain 1 ( ATPC1 ;member of ATP synthase complex) and proton gradient regulation Like 1 ( PGRL1B ;member of CET complex), after 24 h treatment under AL ( P < 0.05; Fig 3B ). ATPC1 transcription significantly increased after 24 h treatment under RL ( P < 0.05; Fig 3B ). No significant difference, after 24 h treatment, was observed in the transcription level of the selected marker genes associated with linear photosynthetic electron transfer (i.e., ferredoxin-2 ( Fd2) , plastocyanin (PETE1) , and cytochrome b6f complex ( PETC ) under both AL and RL ( Fig 3B ). After the 24 h treatment, transcription of ferredoxin-NADP+-oxidoreductase (FNR2) was significantly decreased under AL ( P < 0.05; Fig 3B ), while it remained unchanged under RL ( Fig 3B ). The transcription level of ribulose bisphosphate carboxylase small chain (RBCS1A) was significantly reduced at 2 h and 4 h treatment under both AL and RL ( P < 0.05; Fig 3B ). The RBCS1A transcription level significantly was downregulated under AL ( P < 0.05; Fig 3B ). However, RBCS1A transcription level recovered after the 24 h treatment under RL ( Fig 3B ).
(A) Genes of interest are highlighted in green. (B) Transcription of genes implicated in the light-responsive photosynthetic process that is located within the thylakoid membrane. A time course assessment prior to treatment (0 h), and after treatment (2, 4, and 24 h) of AL and RL was performed, compared to FL. Four biological replicates were examined. For each biological replicate, five A . thaliana plants were selected, and their leaves were pooled together to represent a biological replicate. Plants in each biological replicate were grown independently, and at different time.
https://doi.org/10.1371/journal.pone.0247380.g003
All data were normalized to the housekeeping genes; gamma tonoplast intrinsic protein 2 ( TIP2 ; AT3g26520) and actin 2 ( ACT2 ; AT3g18780). Red borders represent significant changes in expression ( P < 0.05). Studied genes include: ATP synthase gamma chain 1, ATPC1 (AT4g04640); fatty acid desaturase 6, FAD6 (AT4g30950); ferredoxin-2, Fd2 (AT1g60950); ferredoxin-NADP+-oxidoreductase, FNR2 (AT1g20020); (Fdx)-thioredoxin (Trx)-reductase, FTRB (AT2g04700); glutathione synthetase, GSH2 (AT5g27380); PSII nonphotochemical quenching, NPQ1 (AT1g08550); cytochrome b6f complex (Cyt b6f), PETC (AT4g03280); plastocyanin, PETE1 (AT1g76100); proton gradient regulation Like 1, PGRL1B (AT4g11960); photosystem II protein D1, PSBA (ATCG00020) and ribulose bisphosphate carboxylase small chain, RBCS1A (AT1g67090).
To confirm changes in the ATP synthase and CET complex under AL, we leveraged available proteomics data where eleven-leaves plants of A . thaliana Col-0 were grown under AL and RL for 5 days. Consistent with the observed transcriptomic data, a significant increase in the level of protein abundance was observed for both CET complex ( P < 1.3 x 10 −12 ; S1A Fig ) and ATP synthase ( P < 2 x 10 −4 ; S1B Fig ) under AL compared to RL.
Regulation patterns of PSBA , NPQ1 , GSH2 and FAD6 transcripts in A . thaliana Col-0 under AL and RL
The transcription level of photosystem II protein D1 ( PSBA) was significantly upregulated at 4 h and 24 h treatment under RL ( P < 0.05; Fig 3B ). Under AL, the transcription level of PBSA showed a similar increase after the 4 h treatment ( P < 0.05; Fig 3B ); However, its transcription level was reduced to a comparable level with FL after the 24 h treatment under AL. After the 24 h treatment, the transcription level of PSII nonphotochemical quenching (NPQ1) was significantly downregulated under AL ( P < 0.05; Fig 3B ), while it remained steady under RL. Between the 2 h and 4 h treatment, the transcription level of GSH2 gradually increased under both AL and RL ( P < 0.05; Fig 3B ) but reduced to a comparable level with FL after the 24 h treatment under RL. No significant difference was observed in the transcription level of fatty acid desaturase 6 ( FAD6) after the 24 h treatment under either AL or RL ( Fig 3B ).
Photosynthates content in A . thaliana Col-0 under AL and RL
Photosynthates accumulation was probed in Col-0 treated under AL, RL, and FL. Total lipid, protein and starch were measured at days 0, 1, 3, 5, and 7 ( Fig 4 ). The lipid content gradually increased under AL and RL ( P < 0.05; Fig 4A ). The content level of proteins and starches increased under RL but decreased under AL ( P < 0.05; Fig 4B and 4C ).
(A) Lipid; (B) Protein; (C) Starch. Data are expressed as mean values ± standard deviation (n = 5). Statistical analysis was performed against FL using a two-tailed Student’s t-test.
https://doi.org/10.1371/journal.pone.0247380.g004
Antioxidative enzyme activity in A . thaliana Col-0 under AL and RL
We examined the antioxidative activity of superoxide dismutase (SOD) and ascorbate peroxidase (APX) enzymes in Col-0 treated under AL, RL, and FL ( Fig 5 ). After the 24 h treatments, activity of both antioxidants was significantly increased under AL ( P < 0.05; Fig 5 ), while no significant changes were observed for either of these enzymes when plants were treated under RL.
(A) Superoxide dismutase (SOD) activity. One unit of SOD activity was defined as the amount of enzyme required to result in a 50% inhibition of the rate of reduction at 550 nm in 1 min. (B) Ascorbate peroxidase (APX) activity. One unit of APX activity was defined as the amount of enzyme required to oxidize 1 μmol of ascorbate at 290 nm in 1 min. Enzymatic activity was measured for 5 min at room temperature and data are expressed as mean values ± standard deviation (n = 5). Statistical analysis was performed against FL using a two-tailed Student’s t-test (n.s., not statistically significant; *, P < 0.05; **, P < 0.01).
https://doi.org/10.1371/journal.pone.0247380.g005
In this work, we investigated the impact of light quality BL, AL, and RL on leaf growth and photosynthetic response across three A . thaliana accessions Col-0, Est-1, and C24. The analyses clearly demonstrate the significant impact of light quality on leaf area growth, biomass content, and pigments accumulation (chlorophylls, carotenoid, and anthocyanin). The results indicate that light quality significantly influences Pn across accessions, consistent with the reported results that leaf photosynthetic reaction is wavelength-dependent in higher plants [ 54 ].
Importance of geographic habitats on light quality response of leaf growth and biomass
The selected accessions Col-0, Est-1, and C24 have different geographic habitats; C24 originated from a part of Europe (Portugal), Est-1 from Northern Asia (Russia), and Col-0 from United States (Columbia). Therefore, we took into account differences in geographical range for these accessions resulted in a high degree of divergence in photosynthetic characteristics to light [ 35 ]. The most extreme responder in leaf area growth and leaf biomass analyses was Est-1 from Russia. It is worth pointing out that the two Col-0 and C24 accessions highlighted here as weak responders, they elongated very quickly under AL and thus may not be true candidates for weak responders. Previous studies have found negative correlations between hypocotyl height and latitude of accession origin in European Arabidopsis accessions [ 55 , 56 ], suggesting that this natural variation in light sensitivity could be a result of adaptation to the north-south gradient in ambient light intensity.
The results of the study described here emphasize the strength of explicitly incorporating LxG interactions into the leaf area growth and leaf biomass content across the accessions. Importantly, as further elaborated below, the genotype-specific responses in leaf area growth and biomass content were observed exclusively under AL and BL, while the three accessions exhibited similar patterns of changes under RL. Our findings are consistent with previous reports on different accessions and light quality treatments, and underscores the importance of considering the natural habitat effect in characterizing the impact of light quality on leaves [ 57 ].
Leaf development varied between accessions such that the overall dynamic of growth and biomass were different. For example, we took efforts in synchronizing leaf growth stage in the accessions, resulting in the C24 plants being grow for 23 days to reach the same leaf stages of the plant. Some of the observed variation in leaf growth response could be simply a manifestation of the different time-course between accessions. These differences between accession can be significant and have the potential to enhance our understanding of the ecological role of specific adaptations.
Findings on BL supports its role on activation of protective pigments
BL induced higher leaf area growth across three accessions. However, its impact on biomass production is accession-dependent, and may be caused by accessory pigment accumulation (anthocyanins). Under BL, only Col-0 showed an increase in biomass, as opposed to Est-1 and C24, which showed a decrease in biomass. It was observed that BL induced a significantly higher concentration of anthocyanins in Est-1 and C24 than Col-0. These results imply that the impact of wavelength on accessory pigment accumulation is accession-dependent, and that this difference in accessory pigment accumulation consequently leads to differences in biomass production across accessions. Anthocyanin is a photo-protective pigment, which protects plant and its chloroplast membrane by absorbing blue light and against photo-oxidation [ 58 , 59 ]. Higher concentration of anthocyanin accumulating in a plant results in lower BL interception, which consequently lead to lower biomass production over the long term. Further to this, in this study, we found the ratio of Chl a:b is similar under BL and FL across accessions. This consistency in Chl a:b, suggests a lack of photosystems reconfiguration under BL [ 60 , 61 ]. Our results confirm the role of BL in stimulating anthocyanin content in plants and protecting them from light stress [ 62 ]. Plants activate photo-protective mechanisms under BL to cope with a potential induced-light stress, resulting in an increased accumulation of photo-protective pigments [ 58 , 59 ]. Notably, we found different patterns in content of anthocyanin accumulation in the accessions. Results showed that anthocyanin accumulation can be triggered at low BL (~70 μmol m -2 sec -1 ), which suggests that this protective mechanism against BL can vary based on the accession (i.e. natural adaptations) and can be triggered under low light. Further investigation on these two accessions on BL with a wide range of BL intensity is required. Our results thus encourage future studies analyzing this trait using BL with a wide range of BL intensity to further advance our understanding of the underlying mechanisms.
Plants showed high antioxidative and photo-protective under AL
AL had no impact on the photosynthetic activity across the three accessions compared to FL; yet it induced the poorest morphological traits. Col-0 and C24 showed a severe reduction in leaf area growth and biomass, while Est-1 was unaffected. These two accessions (Col-0 and C24) showed a clear elongation of petioles under AL, which suggests that leaf resources are redirected from leaves to petioles as insufficient lighting conditions under AL were performed in this study [ 63 ]. However, the results on transcriptional changes and photosynthates content showed the opposite responses to the morphological traits.
The photosynthates, including proteins and starches, showed lower content in leaves of plants treated under AL. A downregulation of RBCS1A (small subunit of Rubisco) transcription was also observed in the leaves treated under AL. A lower accumulation of proteins was previously observed under AL [ 64 ], suggesting a positive contribution of downregulated Rubisco genes, as it is the main protein in leaves. A lower content of carbohydrates under stress conditions has been observed before in A . thaliana [ 65 ]. Future work is needed to explore if a reduced conversion of light energy into chemical energy has occurred in the photosynthesis process under AL.
High capacity for lipids accumulation was observed for plants treated under AL. Lipid accumulation had been previously linked to oxidative stress [ 66 ]. suggesting an increase in lipophilic antioxidants content such as tocopherols, which play an important role in the scavenging of singlet oxygen [ 67 ]. Moreover, we found a significant increase in both expression and enzymatic activity of antioxidants under AL. Plants stimulate antioxidative mechanisms to protect the photosynthetic apparatus from incurring damage via ROS detoxification [ 30 , 68 ]. Our results on photosynthates thus suggest that plants tried to cope with a potential ROS stress condition under AL.
A significant upregulation in glutathione biosynthesis, transcription level of PGRL1B , ATPC1 , and marker genes associated with ATP synthase and CET complex was observed. In agreement with this result, a significant increase in the expression of ATPC1 at the protein level was recently reported in A . thaliana Col-0 treated with 595 nm light [ 69 ]. CET plays an important role to protect plants under high light and other stress environments [ 70 ]. During CET, electrons are cycled around PSI and protons are translocated to generate a proton gradient across the thylakoid membranes [ 71 ]. In addition to contributing ATP synthesis, another function of a generated proton gradient is to dissipate excess energy as heat from the PSII antennae [ 72 ]. Further to this, an upregulation of CET and ATP synthase suggests of an accelerated rate of PSII repair through elevated ATP synthesis [ 73 , 74 ]. As such, the results on photosynthates and at the transcription level under AL both suggest that AL, even at low light, induces protective mechanisms of photosystems related to light stress, which consequently triggers low protein and starch accumulation and result in poor morphological traits.
One possible hypothesis for the conflicting AL responses can be explained by the detour effect [ 75 , 76 ], where a major part of AL transmitted into the leaf is reflected within leaf tissues and re-absorbed by unsaturated chlorophylls multiple times, which leads to an observed light stress response. Due to the nature of the high absorbing efficiency of the chloroplast, nearly 90% of BL and RL are absorbed at the leaf surface and their detour effect is small [ 76 , 77 ]. While for the wavelength within 500–600 nm [i.e. green light (GL) and AL] that are less absorbed by chloroplast, its light path can increase by several folds and this results in its increased/overexpressed photosynthetic activity through light absorption by unsaturated chloroplast. Although there is no study reporting the underlying mechanisms triggered by AL, several studies have observed the impact of supplemented GL and AL on photosynthetic activity and plant productivity in horticultural plants, which reinforces our hypothesis on the increased photosynthetic activity under AL. Further to this, the aggressive suppression responses on morphological traits in A . thaliana under AL, opposed to the positive impact on plant development, is expected as A . thaliana is a low light plant. They are more sensitive to the change in light properties. Overall, our results suggest AL as a potential light source in activating the potential of increased plant productivity efficiently, but it requires high control on its intensity. This study clarifies why AL alone induces overexpressed high photosynthetic activity yet results in poor plant development.
RL modulated plant adaptation and energy assimilation
The leaf area growth was significantly increased under RL across all accessions, which in turn enabled a greater light interception by the leaves [ 78 ]. This agrees with the increased Pn that was observed across accessions. These observations along with a significant increase of leaf biomass suggests proper plant adaptation under RL across accessions. We found a significant increase in the Chl a: b under RL across accession. Chl a is mainly concentrated around PSI and PSII, whereas Chl b is most abundant in light-harvesting complexes [ 79 ]. An increase in Chl a: b can increase the likelihood of an efficient electron transfer system within the chloroplast membrane [ 80 ]. This, in turn, could positively influence the photosynthetic performance in plants under RL. Considering that timely synthesis of D1 protein is key to maintain the PSII function and consequently, photosynthetic performance in leaves [ 25 ]. An increasing trend of PSBA expression was observed in plants under RL. The PSBA gene is critical for the de novo synthesis of the D1 protein during PSII repairs [ 81 , 82 ]. Therefore, upregulated transcription of PSBA gene could play an important role in accelerating the process of D1 protein turnover under RL. Plants showed that leaf photosynthates (starches, lipids, proteins) increased under RL. Overall, our results present RL as an efficient light source in helping the leaf energy assimilation process, resulting in an increased leaf growth, photosynthetic performance, and photosynthates content in plants.
Supporting information
https://doi.org/10.1371/journal.pone.0247380.s001
S1 Table. List of primers sequences used in qPCR experiments.
https://doi.org/10.1371/journal.pone.0247380.s002
S1 Fig. Proteins involved in ATP synthase and CET complex of A . thaliana Col-0 are upregulated under AL (595 nm) compared to RL (650 nm).
In this experiment, eleven-leaves plants were grown under AL and RL for 5 days (three biological replicates per light condition). A) The expression pattern of protein members involved in Cyclic electron transfer (CET) complex. B) The expression pattern of protein members involved in ATP synthase complex. Expression levels for each protein is normalized to have mean of zero and standard deviation of one. Yellow or blue color indicates upregulation or downregulation, respectively.
https://doi.org/10.1371/journal.pone.0247380.s003
- View Article
- PubMed/NCBI
- Google Scholar
- 31. <oj/>E. W. Schwieterman, "Surface and temporal biosignatures," arXiv preprint arXiv:1803.05065, 2018.
- 38. Hoagland D. R. and Arnon D. I., The water-culture method for growing plants without soil . Berkely,: The College of Agriculture, 1950, p. 32 p.
- 54. Hoover W. H., The dependence of carbon dioxide assimilation in a higher plant on wave length of radiation . City of Washington,: The Smithsonian institution, 1937, p. 1 p.
Thank you for visiting nature.com. You are using a browser version with limited support for CSS. To obtain the best experience, we recommend you use a more up to date browser (or turn off compatibility mode in Internet Explorer). In the meantime, to ensure continued support, we are displaying the site without styles and JavaScript.
- View all journals
- Explore content
- About the journal
- Publish with us
- Sign up for alerts
- Published: 22 March 2023
Photosynthesis re-wired on the pico-second timescale
- Tomi K. Baikie ORCID: orcid.org/0000-0002-0845-167X 1 na1 ,
- Laura T. Wey ORCID: orcid.org/0000-0003-2345-0699 2 , 3 na1 ,
- Joshua M. Lawrence ORCID: orcid.org/0000-0002-9250-8690 2 , 4 ,
- Hitesh Medipally 5 ,
- Erwin Reisner ORCID: orcid.org/0000-0002-7781-1616 4 ,
- Marc M. Nowaczyk ORCID: orcid.org/0000-0002-9269-0672 5 , 6 ,
- Richard H. Friend ORCID: orcid.org/0000-0001-6565-6308 1 ,
- Christopher J. Howe ORCID: orcid.org/0000-0002-6975-8640 2 ,
- Christoph Schnedermann ORCID: orcid.org/0000-0002-2841-8586 1 ,
- Akshay Rao ORCID: orcid.org/0000-0003-4261-0766 1 &
- Jenny Z. Zhang ORCID: orcid.org/0000-0003-4407-5621 4
Nature volume 615 , pages 836–840 ( 2023 ) Cite this article
17k Accesses
15 Citations
1617 Altmetric
Metrics details
- Bioenergetics
- Biological physics
- Biophysical chemistry
- Electron transfer
- Photobiology
Photosystems II and I (PSII, PSI) are the reaction centre-containing complexes driving the light reactions of photosynthesis; PSII performs light-driven water oxidation and PSI further photo-energizes harvested electrons. The impressive efficiencies of the photosystems have motivated extensive biological, artificial and biohybrid approaches to ‘re-wire’ photosynthesis for higher biomass-conversion efficiencies and new reaction pathways, such as H 2 evolution or CO 2 fixation 1 , 2 . Previous approaches focused on charge extraction at terminal electron acceptors of the photosystems 3 . Electron extraction at earlier steps, perhaps immediately from photoexcited reaction centres, would enable greater thermodynamic gains; however, this was believed impossible with reaction centres buried at least 4 nm within the photosystems 4 , 5 . Here, we demonstrate, using in vivo ultrafast transient absorption (TA) spectroscopy, extraction of electrons directly from photoexcited PSI and PSII at early points (several picoseconds post-photo-excitation) with live cyanobacterial cells or isolated photosystems, and exogenous electron mediators such as 2,6-dichloro-1,4-benzoquinone (DCBQ) and methyl viologen. We postulate that these mediators oxidize peripheral chlorophyll pigments participating in highly delocalized charge-transfer states after initial photo-excitation. Our results challenge previous models that the photoexcited reaction centres are insulated within the photosystem protein scaffold, opening new avenues to study and re-wire photosynthesis for biotechnologies and semi-artificial photosynthesis.
This is a preview of subscription content, access via your institution
Access options
Access Nature and 54 other Nature Portfolio journals
Get Nature+, our best-value online-access subscription
$29.99 / 30 days
cancel any time
Subscribe to this journal
Receive 51 print issues and online access
$199.00 per year
only $3.90 per issue
Buy this article
- Purchase on Springer Link
- Instant access to full article PDF
Prices may be subject to local taxes which are calculated during checkout
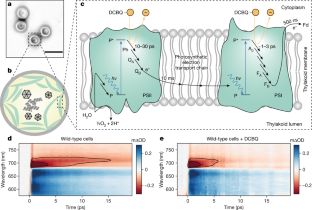
Similar content being viewed by others
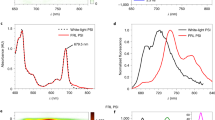
Far-red absorption and light-use efficiency trade-offs in chlorophyll f photosynthesis
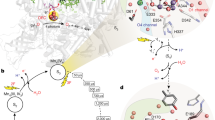
Structural evidence for intermediates during O2 formation in photosystem II
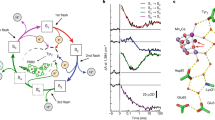
The electron–proton bottleneck of photosynthetic oxygen evolution
Data availability.
The data underlying all figures in the main text are publicly available from the University of Cambridge repository at https://doi.org/10.17863/CAM.92167 .
Code availability
All code used in this work is available from the corresponding authors upon reasonable request.
Grattieri, M., Beaver, K., Gaffney, E. M., Dong, F. & Minteer, S. D. Advancing the fundamental understanding and practical applications of photo-bioelectrocatalysis. Chem. Commun. 56 , 8553–8568 (2020).
Article CAS Google Scholar
Zhang, J. Z. & Reisner, E. Advancing photosystem II photoelectrochemistry for semi-artificial photosynthesis. Nat. Rev. Chem. 4 , 6–21 (2020).
Fu, H.-Y. et al. Redesigning the QA binding site of Photosystem II allows reduction of exogenous quinones. Nat. Commun. 8 , 15274 (2017).
Article PubMed PubMed Central ADS Google Scholar
Jordan, P. et al. Three-dimensional structure of cyanobacterial photosystem I at 2.5 Å resolution. Nature 411 , 909–917 (2001).
Article CAS PubMed ADS Google Scholar
Shen, G., Boussiba, S. & Vermaas, W. F. J. Synechocystis sp PCC 6803 strains lacking photosystem I and phycobilisome function. Plant Cell 5 , 1853 (2007).
Google Scholar
Berera, R., van Grondelle, R. & Kennis, J. T. M. Ultrafast transient absorption spectroscopy: principles and application to photosynthetic systems. Photosynth. Res. 101 , 105–118 (2009).
Article CAS PubMed PubMed Central Google Scholar
Harris, E. H. Chlamydomonas as a model organism. Annu. Rev. Plant Physiol. Plant Mol. Biol. 52 , 363–406 (2001).
Article CAS PubMed Google Scholar
Suda, S. et al. Taxonomic characterization of a marine Nannochloropsis species, N. oceanica sp. nov. (Eustigmatophyceae). Phycologia 41 , 273–279 (2019).
Park, S. et al. Chlorophyll–carotenoid excitation energy transfer and charge transfer in Nannochloropsis oceanica for the regulation of photosynthesis. Proc. Natl Acad. Sci. USA 116 , 3385–3390 (2019).
Article CAS PubMed PubMed Central ADS Google Scholar
Howe, C. J., Barbrook, A. C., Nisbet, R. E. R., Lockhart, P. J. & Larkum, A. W. D. The origin of plastids. Philos. Trans. R. Soc. B: Biol. Sci. 363 , 2675–2685 (2008).
Lea-Smith, D. J. et al. Hydrocarbons are essential for optimal cell size, division, and growth of cyanobacteria. Plant Physiol. 172 , 1928–1940 (2016).
Fălămas, A., Porav, S. A. & Tosa, V. Investigations of the energy transfer in the phycobilisome antenna of Arthrospira platensis using femtosecond spectroscopy. Appl. Sci. 10 , 4045 (2020).
Kopczynski, M. et al. Ultrafast transient lens spectroscopy of various C40 carotenoids: lycopene, β-carotene, (3R,3′ R)-zeaxanthin, (3R,3′ R,6′ R)-lutein, echinenone, canthaxanthin, and astaxanthin. Phys. Chem. Chem. Phys. 7 , 2793–2803 (2005).
Longatte, G. et al. Investigation of photocurrents resulting from a living unicellular algae suspension with quinones over time. Chem. Sci. 9 , 8271–8281 (2018).
Evans, M. C. W. & Heathcote, P. Effects of glycerol on the redox properties of the electron acceptor complex in spinach Photosystem I particles. Biochim. Biophys. Acta Bioenerg. 590 , 89–96 (1980).
De Causmaecker, S., Douglass, J. S., Fantuzzi, A., Nitschke, W. & Rutherford, A. W. Energetics of the exchangeable quinone, Q B , in Photosystem II. Proc. Natl Acad. Sci. USA 116 , 19458–19463 (2019).
Stirbet, A. Excitonic connectivity between photosysstem II units: what is it, and how to measure it? Photosynth. Res. 116 , 189–214 (2013).
Mirkovic, T. et al. Light absorption and energy transfer in the antenna complexes of photosynthetic organisms. Chem. Rev. 117 , 249–293 (2017).
Ma, F., Romero, E., Jones, M. R., Novoderezhkin, V. I. & van Grondelle, R. Both electronic and vibrational coherences are involved in primary electron transfer in bacterial reaction center. Nat. Commun. 10 , 933 (2019).
Dods, R. et al. Ultrafast structural changes within a photosynthetic reaction centre. Nature 589 , 310–314 (2021).
Trebst, A. The three-dimensional structure of the herbicide binding niche on the reaction center polypeptides of photosystem II. Z. Naturforsch. C J. Biosci. 42 , 742–750 (1987).
Longatte, G. et al. Evaluation of photosynthetic electrons derivation by exogenous redox mediators. Biophys. Chem. 205 , 1–8 (2015).
O’Reilly, J. E. Oxidation-reduction potential of the ferro-ferricyanide system in buffer solutions. Biochim. Biophys. Acta Bioenerg. 292 , 509–515 (1973).
Article Google Scholar
Durrant, J. R. et al. Subpicosecond equilibration of excitation energy in isolated photosystem II reaction centers. Proc. Natl Acad. Sci. USA 89 , 11632–11636 (1992).
Groot, M. L. et al. Initial electron donor and acceptor in isolated Photosystem II reaction centers identified with femtosecond mid-IR spectroscopy. Proc. Natl Acad. Sci. USA 102 , 13087–13092 (2005).
Klug, D. R., Durrant, J. R. & Barber, J. The entanglement of excitation energy transfer and electron transfer in the reaction centre of photosystem II. Philos. Trans. R. Soc. London, Ser. A 356 , 449–464 (1998).
Article CAS ADS Google Scholar
Russo, M., Casazza, A. P., Cerullo, G., Santabarbara, S. & Maiuri, M. Ultrafast excited state dynamics in the monomeric and trimeric photosystem I core complex of Spirulina platensis probed by two-dimensional electronic spectroscopy. J. Chem. Phys. 156 , 164202 (2022).
Slavov, C., Ballottari, M., Morosinotto, T., Bassi, R. & Holzwarth, A. R. Trap-limited charge separation kinetics in higher plant photosystem I complexes. Biophys. J. 94 , 3601–3612 (2008).
Lee, Y., Gorka, M., Golbeck, J. H. & Anna, J. M. Ultrafast energy transfer involving the red chlorophylls of cyanobacterial photosystem I probed through two-dimensional electronic spectroscopy. J. Am. Chem. Soc. 140 , 11631–11638 (2018).
Shelaev, I. V. et al. Femtosecond primary charge separation in Synechocystis sp. PCC 6803 photosystem I. Biochim. Biophys. Acta Bioenerg. 1797 , 1410–1420 (2010).
Weliwatte, N. S., Grattieri, M. & Minteer, S. D. Rational design of artificial redox-mediating systems toward upgrading photobioelectrocatalysis. Photochem. Photobiol. Sci. 20 , 1333–1356 (2021).
Bennett, T. et al. Elucidating the role of methyl viologen as a scavenger of photoactivated electrons from photosystem I under aerobic and anaerobic conditions. Phys. Chem. Chem. Phys. 18 , 8512–8521 (2016).
Wey, L. T. et al. The development of biophotovoltaic systems for power generation and biological analysis. Chem. Electro. Chem. 6 , 5375–5386 (2019).
CAS PubMed Google Scholar
Kato, M., Zhang, J. Z., Paul, N. & Reisner, E. Protein film photoelectrochemistry of the water oxidation enzyme photosystem II. Chem. Soc. Rev. 43 , 6485–6497 (2014).
Lea-Smith, D. J., Bombelli, P., Vasudevan, R. & Howe, C. J. Photosynthetic, respiratory and extracellular electron transport pathways in cyanobacteria. Biochim. Biophys. Acta, Bioenerg. 1857 , 247–255 (2016).
Kurashov, V. et al. Critical evaluation of electron transfer kinetics in P700–FA/FB, P700–FX, and P700–A1 Photosystem I core complexes in liquid and in trehalose glass. Biochim. Biophys. Acta Bioenerg. 1859 , 1288–1301 (2018).
Setif, P. Q. Y. & Bottin, H. Laser flash absorption spectroscopy study of ferredoxin reduction by photosystem I: spectral and kinetic evidence for the existence of several photosystem I-ferredoxin complexes. Biochemistry 34 , 9059–9070 (1995).
Young, I. D. et al. Structure of photosystem II and substrate binding at room temperature. Nature 540 , 453–457 (2016).
Stanier, R. Y., Kunisawa, R., Mandel, M. & Cohen-Bazire, G. Purification and properties of unicellular blue-green algae (order Chroococcales). Bacteriol. Rev. 35 , 171–205 (1971).
Lea-Smith, D. J. et al. Thylakoid terminal oxidases are essential for the cyanobacterium Synechocystis sp. PCC 6803 to survive rapidly changing light intensities. Plant Physiol. 162 , 484–495 (2013).
Lea-Smith, D. J. et al. Phycobilisome-deficient strains of Synechocystis sp PCC 6803 have reduced size and require carbon-limiting conditions to exhibit enhanced productivity. Plant Physiol. 165 , 705–714 (2014).
Zhang, J. Z. et al. Photoelectrochemistry of photosystem II in vitro vs in vivo. J. Am. Chem. Soc. 140 , 6–9 (2018).
Mersch, D. et al. Wiring of photosystem II to hydrogenase for photoelectrochemical water splitting. J. Am. Chem. Soc. 137 , 8541–8549 (2015).
El-Mohsnawy, E. et al. Structure and function of intact photosystem 1 monomers from the cyanobacterium Thermosynechococcus elongatus . Biochemistry 49 , 4740–4751 (2010).
Paul, N. Intermolecular Photophysics of Photosystem II Core Complexes at Protein-Nanomaterial Interfaces. PhD thesis, Univ. Cambridge (2015).
Longatte, G., Rappaport, F., Wollman, F.-A., Guille-Collignon, M. & Lemaître, F. Mechanism and analyses for extracting photosynthetic electrons using exogenous quinones–what makes a good extraction pathway? Photochem. Photobiol. Sci. 15 , 969–979 (2016).
Pandya, R., MacQueen, R. W., Rao, A. & Davies, N. J. L. K. Simple and robust panchromatic light harvesting antennacomposites via FRET engineering in solid state host matrices. J. Phys. Chem. C. 122 , 22330–22338 (2018).
Hinrichsen, T. F. et al. Long-lived and disorder-free charge transfer states enable endothermic charge separation in efficient non-fullerene organic solar cells. Nat Commun. 11 , 5617 (2020).
Wey, L. T. et al. A biophotoelectrochemical approach to unravelling the role of cyanobacterial cell structures in exoelectrogenesis. Electrochim. Acta 395 , 139214 (2021).
Download references
Acknowledgements
We acknowledge W. Vermaas (Arizona State University, USA) for the gift of the photosystem-less mutants used in this study and W. Rutherford (Imperial College London) for the gift of isolated PSII as well as valuable discussions on this project. We thank X. Chen for provision of porous electrodes. We acknowledge F. Lemaitre (École Normale Supérieure, France) and P. Rich (University College of London, UK) for helpful discussions about exogenous benzoquinones and photosynthetic microorganisms. We thank K. Redding for helpful discussions on photoexcited states of reaction centre proteins. C.S. and T.K.B. thank V. Gray for insightful discussion at the start of the project. We acknowledge N. Paul for his PhD work, which contributed ideas to this study. T.K.B. gives thanks to the Centre for Doctoral Training in New and Sustainable Photovoltaics (grant no. EP/L01551X/2) and the NanoDTC (grant no. EP/L015978/1) for financial support. L.T.W. acknowledges financial support from the Cambridge Trust. C.S. acknowledges financial support by the Royal Commission of the Exhibition of 1851. We acknowledge financial support by the BBSRC (grant no. BB/R011923/1 to J.Z.Z.), the EPSRC (grant no. EP/M006360/1) and the Winton Program for the Physics of Sustainability as well as from the Deutsche Forschungsgemeinschaft within the framework of the Research Training Group 2341 ‘MiCon’. This project has received funding from the European Research Council under the European Union’s Horizon 2020 research and innovation programme (grant agreement nos. 758826, 764920 and 682833).
Author information
These authors contributed equally: Tomi K. Baikie, Laura T. Wey
Authors and Affiliations
Cavendish Laboratory, University of Cambridge, Cambridge, UK
Tomi K. Baikie, Richard H. Friend, Christoph Schnedermann & Akshay Rao
Department of Biochemistry, University of Cambridge, Cambridge, UK
Laura T. Wey, Joshua M. Lawrence & Christopher J. Howe
Department of Life Technologies, University of Turku, Turku, Finland
Laura T. Wey
Yusuf Hamied Department of Chemistry, University of Cambridge, Cambridge, UK
Joshua M. Lawrence, Erwin Reisner & Jenny Z. Zhang
Plant Biochemistry, Ruhr University Bochum, Bochum, Germany
Hitesh Medipally & Marc M. Nowaczyk
Department of Biochemistry, University of Rostock, Rostock, Germany
Marc M. Nowaczyk
You can also search for this author in PubMed Google Scholar
Contributions
T.K.B. and L.T.W. contributed equally to the work and initially developed the application of ultrafast techniques to exame cyanobacteria. C.S. and A.R. supervised the spectroscopy, C.J.H. supervised the cell work, J.Z.Z. developed the research question. T.K.B. performed the TA and TCSPC experiments and the analysis, and prepared the figures. L.T.W. chose and prepared the samples for TA and TCSPC, performed the photo-electrochemistry, oxygen evolution, cytotoxicity and microscopy experiments, and did protein crystal structure analysis. J.M.L. prepared samples for the MV 2+ study. H.M. prepared isolated PSI. T.K.B., L.T.W., M.M.N., R.H.F., E.R., C.S., J.Z.Z., C.J.H. and A.R. contributed to discussions, analysis and writing of the manuscript.
Corresponding authors
Correspondence to Christopher J. Howe , Christoph Schnedermann , Akshay Rao or Jenny Z. Zhang .
Ethics declarations
Competing interests.
The authors declare no competing interests.
Peer review
Peer review information.
Nature thanks the anonymous reviewers for their contribution to the peer review of this work. Peer reviewer reports are available.
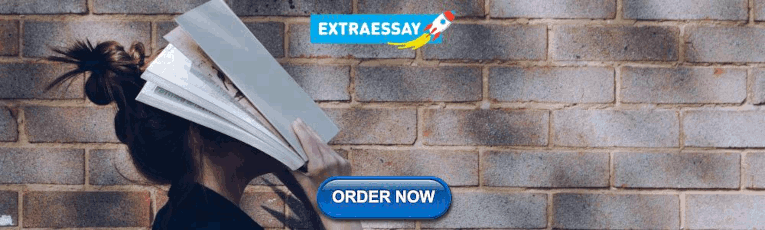
Additional information
Publisher’s note Springer Nature remains neutral with regard to jurisdictional claims in published maps and institutional affiliations.
Supplementary information
Supplementary information.
This file contains Supplementary Figs. 1–32 and Tables 1–12.
Peer Review File
Rights and permissions.
Springer Nature or its licensor (e.g. a society or other partner) holds exclusive rights to this article under a publishing agreement with the author(s) or other rightsholder(s); author self-archiving of the accepted manuscript version of this article is solely governed by the terms of such publishing agreement and applicable law.
Reprints and permissions
About this article
Cite this article.
Baikie, T.K., Wey, L.T., Lawrence, J.M. et al. Photosynthesis re-wired on the pico-second timescale. Nature 615 , 836–840 (2023). https://doi.org/10.1038/s41586-023-05763-9
Download citation
Received : 31 January 2022
Accepted : 26 January 2023
Published : 22 March 2023
Issue Date : 30 March 2023
DOI : https://doi.org/10.1038/s41586-023-05763-9
Share this article
Anyone you share the following link with will be able to read this content:
Sorry, a shareable link is not currently available for this article.
Provided by the Springer Nature SharedIt content-sharing initiative
This article is cited by
Bioinspired polymeric supramolecular columns as efficient yet controllable artificial light-harvesting platform.
- Xiangnan Hao
Nature Communications (2024)
Directed ultrafast conformational changes accompany electron transfer in a photolyase as resolved by serial crystallography
- Andrea Cellini
- Madan Kumar Shankar
- Sebastian Westenhoff
Nature Chemistry (2024)
Rewiring photosynthetic electron transport chains for solar energy conversion
- Joshua M. Lawrence
- Rachel M. Egan
- Jenny Z. Zhang
Nature Reviews Bioengineering (2023)
By submitting a comment you agree to abide by our Terms and Community Guidelines . If you find something abusive or that does not comply with our terms or guidelines please flag it as inappropriate.
Quick links
- Explore articles by subject
- Guide to authors
- Editorial policies
Sign up for the Nature Briefing newsletter — what matters in science, free to your inbox daily.

- Search Menu
- Advance Articles
- Editor's Choice
- Commentaries
- Special Issues
- Rapid Papers
- Author Guidelines
- Submission Site
- Open Access Options
- Author Benefits
- Benefits of Publishing Open Access
- About Plant and Cell Physiology
- About the Japanese Society of Plant Physiologists
- Editorial Board
- Permissions
- Advertising and Corporate Services
- Journals Career Network
- Self-Archiving Policy
- Journals on Oxford Academic
- Books on Oxford Academic
Article Contents
Photosynthetic research in plant science.
- Article contents
- Figures & tables
- Supplementary Data
Ayumi Tanaka, Amane Makino, Photosynthetic Research in Plant Science, Plant and Cell Physiology , Volume 50, Issue 4, April 2009, Pages 681–683, https://doi.org/10.1093/pcp/pcp040
- Permissions Icon Permissions
Photosynthesis is a highly regulated, multistep process. It encompasses the harvest of solar energy, transfer of excitation energy, energy conversion, electron transfer from water to NADP + , ATP generation and a series of enzymatic reactions that assimilate carbon dioxide and synthesize carbohydrate.
Photosynthesis has a unique place in the history of plant science, as its central concepts were established by the middle of the last century, and the detailed mechanisms have since been elucidated. For example, measurements of photosynthetic efficiency (quantum yield) at different wavelengths of light (Emerson and Lews 1943 ) led to the insight that two distinct forms of Chl must be excited in oxygenic photosynthesis. These results suggested the concept of two photochemical systems. The reaction center pigments of PSII and PSI (P680 and P700, respectively) were found by studying changes in light absorbance in the red region (Kok 1959 , Döring et al. 1969 ). Chls with absorbance maxima corresponding to these specific wavelengths were proposed as the final light sink. These Chls were shown to drive electron transfer by charge separation. The linkage of electron transfer and CO 2 assimilation was suggested by studies on Hill oxidant (Hill 1937 ). A linear electron transport system with two light-driven reactions (Z scheme) was proposed based upon observations of the redox state of cytochromes (Hill and Bendall 1960 , Duysens et al. 1961 ), and photophosphorylation was found to be associated with thylakoid fragments (Arnon et al. 1954 ). The metabolic pathway that assimilates carbon by fixation of CO 2 was discovered by Calvin's group who used 14 CO 2 radioactive tracers in the 1950s (Bassham and Calvin 1957 ). This was the first significant discovery in biochemistry made using radioactive tracers. The primary reaction of CO 2 fixation is catalyzed by Rubisco (Weissbach et al. 1956 ), initially called Fraction 1 protein (Wildman and Bonner 1947 ). Rubisco is the most abundant protein in the world, largely because it is also the most inefficient with the lowest catalytic turnover rate (1–3 s –1 ). Another CO 2 fixation pathway was then found in sugarcane (Kortschak et al. 1964, Hatch and Slack 1965) and named C 4 photosynthesis.
Although photosynthesis plays the central role in the energy metabolism of plants, historically there have not been strong interactions between photosynthesis research and other fields of plant science. Many techniques and tools developed for photosynthesis research have not been widely used in other fields because they were developed to examine phenomena unique to photosynthesis. For example, excitation energy transfer and charge separation are fundamental but unique processes of photosynthesis. Another reason for the historic isolation of photosynthesis research within plant science is that it was long believed that CO 2 fixation and carbohydrate production are the sole function of photosynthesis, with carbohydrates representing the only link between photosynthesis and other biological phenomena.
However, this situation has begun to change. Recent research has revealed that photosynthesis is closely related to a variety of other physiological processes. It is a major system for controlling the redox state of cells, playing an important role in regulating enzyme activity and many other cellular processes (Buchanan and Balmer 2005 , Hisabori et al. 2007 ). Photosynthesis also generates reactive oxygen species, which are now appreciated as being regulatory factors for many biological processes rather than inevitable by-products of photosynthesis (Wagner et al. 2004 , Beck 2005 ). Precursor molecules of Chl, which are a major component of photosynthesis, act as a chloroplast-derived signal, and are involved in regulating the cell cycle (Kobayashi et al. 2009 ). In light of this new information, it seems important to re-evaluate the function(s), both potential and demonstrated, of photosynthesis from a variety of view points. Photosynthesis research now employs the methods and tools of molecular biology and genetics, which are central methods for plant science in general. Meanwhile, Chl fluorescence and gas exchange measurements, developed especially for photosynthesis research, are now widely used in stress biology and ecology.
Photosynthesis research also contributes to our understanding of ecological phenomena and even the global environments (Farquhar et al. 1980 , de Pury and Farquhar 1997 , Monsi and Saeki 2005 ). Indeed, photosynthesis is now an integral component of simulation models used to predict the future of our planet. Improving the efficiency of photosynthesis by artificial modification of photosynthetic proteins and pathways has long been considered impossible or at best problematic, because, over evolutionary time, photosynthesis has become complex and tightly regulated. However, recent advances have made it possible to manipulate photosynthesis using molecular genetic technology (Andrews and Whiney 2003 , Raines 2006 ). These advances may have positive influences on crop productivity (Parry et al. 2007 ) as photosynthetic rates have frequently been correlated with biomass accretion (Kruger and Volin 2006 ). Thus, we can expect many more novel concepts to be added to this history of photosynthetic research.
As photosynthesis research tackles new challenges, we should also continue to re-evaluate past research. Oxygen evolution, energy dissipation and cyclic electron transport are crucial processes during photosynthesis, yet their mechanisms still remain to be clarified. We have very limited knowledge of the formation and degradation of photosynthetic apparatus. Also, although photosynthesis plays a central role in C and N metabolism in plants, we do not yet understand how potential photosynthesis is related to crop productivity.
Plant and Cell Physiology would like to contribute to the development of novel concepts, pioneering new fields and solving the unanswered questions of photosynthesis. This special issue covers a wide range of topics in photosynthesis research. Terashima et al. (pp. 684–697) readdress the enigmatic question of why leaves are green. They show that the light profile through a leaf is steeper than that of photosynthesis, and that the green wavelengths in white light are more effective in driving photosynthesis than red light. Evans (pp. 698–706) proposes a new model using Chl fluorescence to explore modifications in quantum yield with leaf depth. This new multilayered model can be applied to study variations in light absorption profiles, photosynthetic capacity and calculation of chloroplastic CO 2 concentration at different depths through the leaf.
Singlet oxygen, 1 O 2 , is produced by the photosystem and Chl pigments. 1 O 2 not only causes physiological damage but also activates stress response programs. The flu mutant of Arabidopsis thaliana overaccumulates protochlorophyllide that upon illumination generates singlet oxygen, causing growth cessation and cell death. Coll et al. (pp. 707–718) have isolated suppressor mutants, dubbed ‘singlet oxygen-linked death activator’ (soldat), that specifically abrogate 1 O 2 -mediated stress responses in young flu seedlings, and they discuss the processes of acclimation to stresses. Phephorbide a is a degradation product of Chl and one of the most powerful photosensitzing molecules. Mutants defective in pheophorbide a oxygenase, which converts phephorbide a to open tetrapyrrole, accumulate pheophorbide a and display cell death in a light-dependent manner. Hirashima et al. (pp. 719–729) report that pheophorbide a is involved in this light-independent cell death.
Plants regulate the redox level of the plastoquinone pool in response to the light environment. In acclimation to high-light conditions, the redox level is kept in an oxidized state by the plastoquinone oxidation system (POS). Miyake et al. (pp. 730–743) investigated the mechanism of POS using the Chl fluorescence parameter, qL.
Nagai and Makino (pp. 744–755) examine in detail the differences between rice and wheat, the two most commercially important crops, in the temperature responses of CO 2 assimilation and plant growth. They find that the difference in biomass production between the two species at the level of the whole plant depends on the difference in N-use efficiency in leaf photosynthesis and growth rate. Sage and Sage (pp. 756–772) examine chlorenchyma structure in rice and related Oryza species in relation to photosynthetic function. They find that rice chlorenchyma architecture includes adaptations to maximize the scavenging of photorespired CO 2 and to enhance the diffusive conductance of CO 2 . In addition, they consider that the introduction of Kranz anatomy does not require radical anatomical alterations in engineering C 4 rice.
Bioinformatics has become a powerful tool, especially in photosynthetic research, because photosynthetic organisms have a wide taxonomic distribution among prokaryotes and eukaryotes. Ishikawa et al. (pp. 773–788) present the results of a pilot study of functional orthogenomics, combining bioinformatic and experimental analyses to identify nuclear-encoded chloroplast proteins of endosymbiontic origin (CRENDOs). They conclude that phylogenetic profiling is useful in finding CPRENDOs, although the physiological functions of orthologous genes may be different in chloroplasts and cyanobacteria.
We hope you enjoy this special issue, and would like to invite you to submit more excellent papers to Plant and Cell Physiology in the field of photosynthesis.
Google Scholar
Google Preview
Email alerts
Citing articles via.
- Recommend to Your Librarian
Affiliations
- Online ISSN 1471-9053
- Copyright © 2024 Japanese Society of Plant Physiologists
- About Oxford Academic
- Publish journals with us
- University press partners
- What we publish
- New features
- Open access
- Institutional account management
- Rights and permissions
- Get help with access
- Accessibility
- Advertising
- Media enquiries
- Oxford University Press
- Oxford Languages
- University of Oxford
Oxford University Press is a department of the University of Oxford. It furthers the University's objective of excellence in research, scholarship, and education by publishing worldwide
- Copyright © 2024 Oxford University Press
- Cookie settings
- Cookie policy
- Privacy policy
- Legal notice
This Feature Is Available To Subscribers Only
Sign In or Create an Account
This PDF is available to Subscribers Only
For full access to this pdf, sign in to an existing account, or purchase an annual subscription.
share this!
May 10, 2024
This article has been reviewed according to Science X's editorial process and policies . Editors have highlighted the following attributes while ensuring the content's credibility:
fact-checked
peer-reviewed publication
trusted source
Researchers shed new light on carboxysomes in key discovery that could boost photosynthesis
by Hong Kong University of Science and Technology
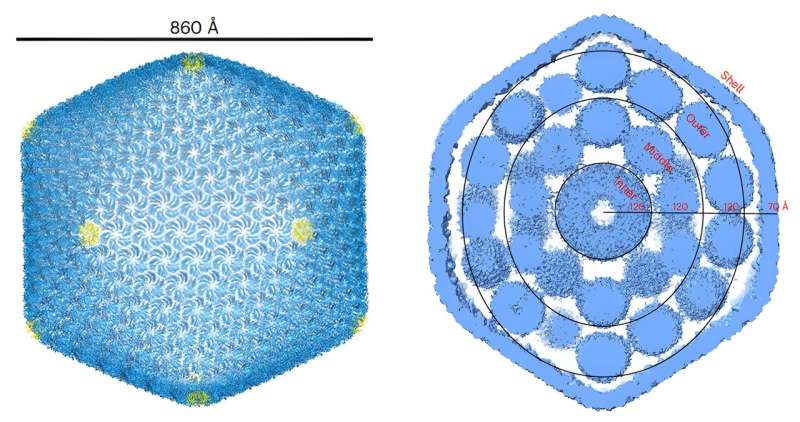
A research team led by the Hong Kong University of Science and Technology (HKUST) has discovered how carboxysomes—carbon-fixing structures found in some bacteria and algae—work. The breakthrough could help scientists redesign and repurpose the structures to enable plants to convert sunlight into more energy, paving the way for improved photosynthesis efficiency, potentially increasing the global food supply and mitigating global warming.
Carboxysomes are tiny compartments in certain bacteria and algae that encase particular enzymes in a shell made of proteins. They perform carbon fixation , which is the process of converting carbon dioxide from the atmosphere into organic compounds that can be used by the cell for growth and energy. Scientists have been trying to figure out how these compartments put themselves together.
In their latest research, the team led by Prof. Zeng Qinglu, Associated Professor at HKUST's Department of Ocean Science, has shown the overall architecture of carboxysomes purified from a type of bacteria called Prochlorococcus.
In collaboration with Prof. Zhou Cong-Zhao of the School of Life Sciences in the University of Science & Technology of China, the team overcame one of the biggest technical difficulties in cell breakage and contamination, which would prevent the proper purification of carboxysomes. The team also proposes a complete assembly model of α-carboxysome, which has not been observed in previous studies.
Their research is published in the journal Nature Plants .
The team specifically utilized single-particle cryo- electron microscopy to determine the structure of α-carboxysome and characterize the assembly pattern of the protein shell, which looks like a 20-sided shape with specific proteins arranged on its surface. To obtain the structure of the minimal α-carboxysome with a diameter of 86 nm, they collected over 23,400 images taken from the microscope at the HKUST Biological Cryo-EM Center and manually picked about 32,000 intact α-carboxysome particles for analysis.
Inside, the RuBisCO enzymes are arranged in three concentric layers, and the research team also discovered that a protein called CsoS2 helps to hold everything together inside the shell. Finally, the findings suggest that carboxysomes are put together from the outside in. This means that the inside surface of the shell is strengthened by certain parts of the CsoS2 protein, while other parts of the protein attract the RuBisCO enzymes and organize them into layers.
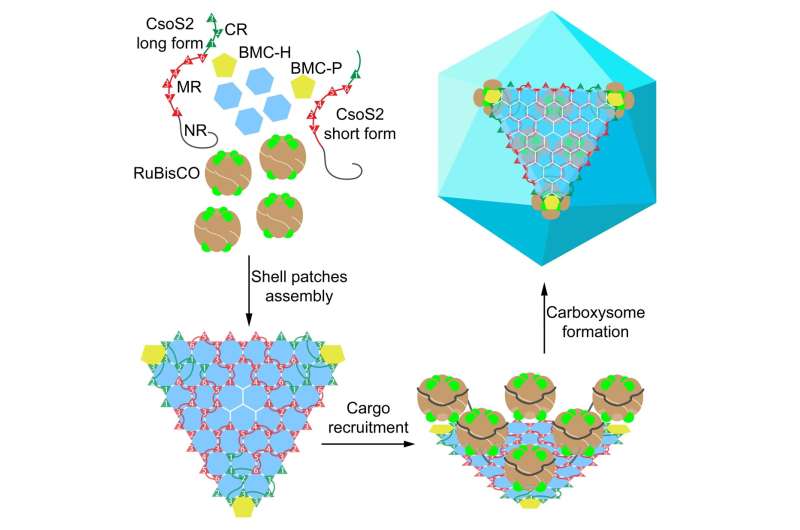
One of the most promising application of carboxysome is in plant synthetic biology , whereby the introduction of carboxysome into plant chloroplasts as the CO 2 -concentrating mechanism can improve photosynthetic efficiency and crop yield.
"Our study unveils the mystery of α-carboxysome assembly from Prochlorococcus, thus providing novel insights into global carbon cycling," says Prof. Zeng.
The findings will also be important to slow down global warming , he explains, as marine cyanobacteria fix 25% of global CO 2 . "Our understanding of the CO 2 fixation mechanism of marine cyanobacteria will enable us to improve their CO 2 fixation rate so that more CO 2 can be removed from the atmosphere," he says.
Following this study, the team plans to introduce Prochlorococcus α-carboxysome into plant chloroplasts and investigate whether the minimal α-carboxysome can improve the photosynthetic efficiency in plants. They also plan to modify the carboxysome genes and make genetically modified super cyanobacteria that are able to fix carbon dioxide at very high rates, which may be able to slow down global warming.
Journal information: Nature Plants
Provided by Hong Kong University of Science and Technology
Explore further
Feedback to editors

Tiger beetles fight off bat attacks with ultrasonic mimicry
5 hours ago

Machine learning model uncovers new drug design opportunities
9 hours ago

Astronomers find the biggest known batch of planet ingredients swirling around young star

How 'glowing' plants could help scientists predict flash drought

New GPS-based method can measure daily ice loss in Greenland
10 hours ago

New candidate genes for human male infertility found by analyzing gorillas' unusual reproductive system
11 hours ago

Study uncovers technologies that could unveil energy-efficient information processing and sophisticated data security

Scientists develop an affordable sensor for lead contamination

Chemists succeed in synthesizing a molecule first predicted 20 years ago

New optical tweezers can trap large and irregularly shaped particles
12 hours ago
Relevant PhysicsForums posts
Is it usual for vaccine injection site to hurt again during infection.
May 13, 2024
A Brief Biography of Dr Virgina Apgar, creator of the baby APGAR test
May 12, 2024
Who chooses official designations for individual dolphins, such as FB15, F153, F286?
May 9, 2024
The Cass Report (UK)
May 1, 2024
Is 5 milliamps at 240 volts dangerous?
Apr 29, 2024
Major Evolution in Action
Apr 22, 2024
More from Biology and Medical
Related Stories

Study reveals how bacteria build essential carbon-fixing machinery
Jul 8, 2020

Cell imaging could provide next step for developing synthetic photosynthesis
Apr 5, 2023

How bacteria build hyper-efficient photosynthesis machines
Jan 24, 2019

How to build an artificial nano-factory to power our futures
Jun 19, 2017

A boost for photosynthesis

Scientists take step to improve crops' photosynthesis, yields
Aug 13, 2021
Recommended for you

Study shows that capuchin monkeys use sticks and stones to dig for food underground
14 hours ago

Scientists unlock mysteries of orangutan communication

High genetic diversity discovered in South African leopards

In a reservoir in Southeast Brazil, introduction of a fish native to the Amazon has reduced native species diversity
Let us know if there is a problem with our content.
Use this form if you have come across a typo, inaccuracy or would like to send an edit request for the content on this page. For general inquiries, please use our contact form . For general feedback, use the public comments section below (please adhere to guidelines ).
Please select the most appropriate category to facilitate processing of your request
Thank you for taking time to provide your feedback to the editors.
Your feedback is important to us. However, we do not guarantee individual replies due to the high volume of messages.
E-mail the story
Your email address is used only to let the recipient know who sent the email. Neither your address nor the recipient's address will be used for any other purpose. The information you enter will appear in your e-mail message and is not retained by Phys.org in any form.
Newsletter sign up
Get weekly and/or daily updates delivered to your inbox. You can unsubscribe at any time and we'll never share your details to third parties.
More information Privacy policy
Donate and enjoy an ad-free experience
We keep our content available to everyone. Consider supporting Science X's mission by getting a premium account.
E-mail newsletter
Advertisement
Photosynthesis research under climate change
- Published: 07 July 2021
- Volume 150 , pages 5–19, ( 2021 )
Cite this article
- Sajad Hussain ORCID: orcid.org/0000-0001-9100-360X 1 , 2 na1 ,
- Zaid Ulhassan 3 na1 ,
- Marian Brestic ORCID: orcid.org/0000-0003-3470-6100 4 ,
- Marek Zivcak 4 ,
- Weijun Zhou 3 ,
- Suleyman I. Allakhverdiev ORCID: orcid.org/0000-0002-0452-232X 5 ,
- Xinghong Yang 6 ,
- Muhammad Ehsan Safdar ORCID: orcid.org/0000-0002-1865-5182 7 ,
- Wenyu Yang 1 , 2 &
- Weiguo Liu ORCID: orcid.org/0000-0002-1804-0276 1 , 2
9866 Accesses
68 Citations
2 Altmetric
Explore all metrics
Increasing global population and climate change uncertainties have compelled increased photosynthetic efficiency and yields to ensure food security over the coming decades. Potentially, genetic manipulation and minimization of carbon or energy losses can be ideal to boost photosynthetic efficiency or crop productivity. Despite significant efforts, limited success has been achieved. There is a need for thorough improvement in key photosynthetic limiting factors, such as stomatal conductance, mesophyll conductance, biochemical capacity combined with Rubisco, the Calvin–Benson cycle, thylakoid membrane electron transport, nonphotochemical quenching, and carbon metabolism or fixation pathways. In addition, the mechanistic basis for the enhancement in photosynthetic adaptation to environmental variables such as light intensity, temperature and elevated CO 2 requires further investigation. This review sheds light on strategies to improve plant photosynthesis by targeting these intrinsic photosynthetic limitations and external environmental factors.
This is a preview of subscription content, log in via an institution to check access.
Access this article
Price excludes VAT (USA) Tax calculation will be finalised during checkout.
Instant access to the full article PDF.
Rent this article via DeepDyve
Institutional subscriptions
Similar content being viewed by others
Impact of Changing Abiotic Environment on Photosynthetic Adaptation in Plants
The Multifaceted Connections Between Photosynthesis and Respiratory Metabolism
Strategies for Engineering Photosynthesis for Enhanced Plant Biomass Production
Adachi S, Nakae T, Uchida M, Soda K, Takai T, Oi T et al (2013) The mesophyll anatomy enhancing CO2 diffusion is a key trait for improving rice photosynthesis. J Exp Bot 64:1061–1072
CAS PubMed Google Scholar
Ainsworth EA, Long SP (2005) What have we learned from 15 years of free-air CO 2 enrichment (FACE)? A meta-analytic review of the responses of photosynthesis, canopy properties and plant production to rising CO2. New Phytol 165:351–371
PubMed Google Scholar
Ainsworth EA, Davey PA, Bernacchi CJ, Dermody OC, Heaton EA, Moore DJ, Morgan PB, Naidu SL, Yoo Ra HS, Zhu XG, Curtis PS (2002) A meta analysis of elevated [CO2] effects on soybean (Glycine max) physiology, growth and yield. Glob Change Biol 8(8):695–709
Google Scholar
Atkinson N, Mao Y, Chan KX, McCormick AJ (2020) Condensation of Rubisco into a proto-pyrenoid in higher plant chloroplasts. Nat Commun 11(1):1–9
Bathellier C, Tcherkez G, Lorimer GH, Farquhar GD (2018) Rubisco is not really so bad. Plant Cell Environ 41(4):705–716. https://doi.org/10.1111/pce.13149
Article CAS PubMed Google Scholar
Batista-Silva W, da Fonseca-Pereira P, Martins AO, Zsogon A, Nunes-Nesi A, Araujo WL (2020) Engineering improved photosynthesis in the era of synthetic biology. Plant Commun 1: 100032
PubMed PubMed Central Google Scholar
Berry JO, Yerramsetty P, Zielinski AM, Mure CM (2013) Photosynthetic gene expression in higher plants. Photosynth Res 117(1):91–120
Bielczynski LW, Schansker G, Croce R (2019) Consequences of the reduction of the PSII antenna size on the light acclimation capacity of Arabidopsis thaliana . Plant Cell Environ Adv Access Publ. https://doi.org/10.1111/pce.13701
Article Google Scholar
Blankenship RE, Tiede DM, Barber J et al (2011) Comparing photosynthetic and photovoltaic efficiencies and recognizing the potential for improvement. Science 332:805–809
Bracher A, Whitney SM, Hartl FU, Hayer-Hartl M (2017) Biogenesis and metabolic maintenance of Rubisco. Ann Rev Plant Bio 68:29–60
CAS Google Scholar
Brestic M, Zivcak M, Hauptvogel P, Misheva S, Kocheva K, Yang X, Allakhverdiev SI (2018) Wheat plant selection for high yields entailed improvement of leaf anatomical and biochemical traits including tolerance to non-optimal temperature conditions. Photosynth Res 136(2):245–255
Brestic M, Zivcak M, Kalaji HM, Carpentier R, Allakhverdiev SI (2012) Photosystem II thermostability in situ: environmentally induced acclimation and genotype-specific reactions in Triticumaestivum L. Plant Physiol Biochem 57:93–105
Buntru M, Gärtner S, Staib L, Kreuzaler F, Schlaich N (2013) Delivery of multiple transgenes to plant cells by an improved version of Multi Round Gateway technology. Trans Res 22(1):153–167
Carmo-Silva, E., Scales, J. C., Madgwick, P. J., & Parry, M. A. (2015) Optimizing R ubisco and its regulation for greater resource use efficiency. Plant Cell Environ 38(9):1817–1832
Cen Y-P, Sage RF (2005) The regulation of rubisco activity in response to variation in temperature and atmospheric CO 2 partial pressure in sweet potato. Plant Physiol 139:979–990
CAS PubMed PubMed Central Google Scholar
Chang H, Huang HE, Cheng CF, Ho MH, Ger MJ (2017) Constitutive expression of a plant ferredoxin-like protein (pflp) enhances capacity of photosynthetic carbon assimilation in rice (Oryza sativa). Transgenic Res 26:279–289
Chang TG, Zhu XG (2017) Source–sink interaction: a century old concept under the light of modern molecular systems biology. J Exp Bot 68:4417–4431
Chen JH, Chen ST, He NY, Wang QL, Zhao Y, Gao W, Guo FQ (2020) Nuclearencoded synthesis of the D1 subunit of photosystem II increases photosynthetic efficiency and crop yield. Nat Plant 6(5):570–580
Chen X, Hao M-D (2015) Low contribution of photosynthesis and water-use efficiency to improvement of grain yield in Chinese wheat. Photosynthetica 53:519–526
Chida H, Nakazawa A, Akazaki H, Hirano T, Suruga K, Ogawa M, SatohT KK, Yamada S, Hakamata W et al (2007) Expression of thealgal cytochrome c6 gene in Arabidopsis enhances photosynthesis andgrowth. Plant Cell Physiol 48:948–957
Cramer WA, Zhang H (2006) Consequences of the structure of the cytochrome b6f complex for its charge transfer pathways. Biochim Biophys Acta 1757:339–345
Dann M, Leister D (2017) Enhancing (crop) plant photosynthesis by introducing novel genetic diversity. Philos Trans R Soc B 372(1730):20160380
Delucia EH, Sasek TW, Strain BR (1985) Photosynthetic inhibition after long-term exposure to elevated levels of atmospheric carbon dioxide. Photosynth Res 7(2):175–184
Demmig-Adams B, Adams WW (2006) Photoprotection in an ecological context: the remarkable complexity of thermal energy dissipation. New Phytol 172:11–21
DePaoli HC, Borland AM, Tuskan GA, Cushman JC, Yang X (2014) Synthetic biology as it relates to CAM photosynthesis: challenges and opportunities. J Exp Bot 65(13):3381–3393
Detto M, Xu X (2020) Optimalleaflifestrategiesdetermine V c, maxdynamicduringontogeny. New Phytol. https://doi.org/10.1111/nph.16712
Article PubMed Google Scholar
Driever SM, Lawson T, Andralojc PJ, Raines CA, Parry MAJ (2014) Natural variation in photosynthetic capacity, growth, and yield in 64 field-grown wheat genotypes. J Exp Bot 65(17):4959–4973. https://doi.org/10.1093/jxb/eru253
Article CAS PubMed PubMed Central Google Scholar
Driever SM, Simkin AJ, Alotaibi S, Fisk SJ, Madgwick PJ, Sparks CA, Raines CA (2017) Increased SBPase activity improves photosynthesis and grain yield in wheat grown in greenhouse conditions. Philos Trans R Soc B 372(1730):20160384
Dwyer SA, Ghannoum O, Nicotra A, von Caemmerer S (2007) High temperature acclimation of C4 photosynthesis is linked to changes in photosynthetic biochemistry. Plant Cell Environ 30:53–66
Eisenhut M, Bräutigam A, Timm S, Florian A, Tohge T, Fernie AR, Bauwe H, Weber APM (2017) photorespiration is crucial for dynamic response of photosynthetic metabolism and stomatal movement to altered CO 2 availability. Mol Plant 10(1):47–61. https://doi.org/10.1016/j.molp.2016.09.011
Emmel C, D’Odorico P, Revill A, Hörtnagl L, Ammann C, Buchmann N, Eugster W (2020) Canopy photosynthesis of six major arable crops is enhanced under diffuse light due to canopy architecture. Glob Change Biol 26(9):5164–5177
Engineer CB, Hashimoto-Sugimoto M, Negi J, Israelsson-Nordström M, Azoulay-Shemer T, Rappel WJ, Iba K, Schroeder JI (2016) CO 2 sensing and CO 2 regulation of stomatal conductance: advances and open questions. Trends Plant Sci 21(1):16–30
Ermakova M, Lopez-Calcagno PE, Raines CA, Furbank RT, von Caemmerer S (2019) Overexpression of the Rieske FeS protein of the Cytochrome b 6 f complex increases C 4 photosynthesis in Setaria viridis. Commun Biol 2(1):1–12
Éva C, Oszvald M, Tamás L (2019) Current and possible approaches for improving photosynthetic efficiency. Plant Sci 280:433–440
Evans JR (2013) Improving photosynthesis. Plant Physiol 162(4):1780–1793
Fankhausen N, Aubry S (2017) Post-transcriptional regulation of photosynthetic genes is a key driver of C4 leaf ontogeny. J Exp Bot 68:137–146
Fernie AR, Bachem CW, Helariutta Y, Neuhaus HE, Prat S, Ruan YL, Sonnewald U (2020) Synchronization of developmental, molecular and metabolic aspects of source–sink interactions. Nat Plants 6(2):55–66
Fernie AR, Yan J (2019) De novo domestication: an alternative route toward new crops for the future. Mol Plant 12(5):615–631
Flecken M, Wang H, Popilka L, Hartl FU, Bracher A, Hayer-Hartl M (2020) Dual role of a rubisco activase in metabolic repair and carboxysome organization. BioRxiv.
Flexas J, Ribas-Carbo M, Diaz-Espejo A, Medrano H (2008) Mesophyll conductance to CO 2 : current knowledge and future prospects. Plant Cell Environ 31:602–621
Flöck D, Helms V (2004) A Brownian dynamics study: the effect of a membrane environment on an electron transfer system. Biophysical J 87(1):65–74
Foyer CH, Shigeoka S (2011) Understanding oxidative stress and antioxidant functions to enhance photosynthesis. Plant Physiol 155(1):93–100
Franks PJ, Doheny-Adams T, Britton-Harper ZJ, Gray JE (2015) Increasing water use efficiency directly through genetic manipulation of stomatal density. New Phytol 207:188–195
Furbank RT, Sharwood R, Estavillo GM, Silva-Perez V, Condon AG (2020) Photons to food: genetic improvement of cereal crop photosynthesis. Jexp Bot 71(7):2226–2238
Gago J, Daloso DM, Carriquí M, Nadal M, Morales M, Araújo WL, Nunes-Nesi A, Flexas J (2020) Mesophyll conductance: the leaf corridors for photosynthesis. Biochem Soc Trans 48(2):429–439
Gong HY, Li Y, Fang G, Hu DH, Jin WB, Wang ZH, Li YS (2015) Transgenic rice expressing Ictb and FBP/Sbpase derived from cyanobacteria exhibits enhanced photosynthesis and mesophyll conductance to CO2. PLoS ONE 10: e0140928
Gu J, Zhou Z, Li Z, Chen Y, Wang Z, Zhang H (2017) Rice ( Oryza sativa L.) with reduced chlorophyll content exhibit higher photosynthetic rate and efficiency, improved canopy light distribution, and greater yields than normally pigmented plants. Field Crop Res 200:58–70
Gutierrez-Rodriquez M et al (2000) Photosynthesis of wheat in a warm, irrigated environment II. Traits associated with genetic gains in yield. Field Crops Res 66:51–62
Guy RD, Momayyezi M, McKown AD, Bell SCS (2019) Emerging roles for carbonic anhydrase in mesophyll conductance and photosynthesis. Plant J 101:831–844
Hay WT, Bihmidine S, Mutlu N, Le Hoang K, Awada T, Weeks DP, Long SP (2017) Enhancing soybean photosynthetic CO2 assimilation using a cyanobacterial membrane protein, ictB. J Plant Physiol 212:58–68
Hendrickson L, Sharwood R, Ludwig M, Whitney SM, Badger MR, von Caemmerer S (2008) The effects of Rubisco activase on C4 photosynthesis and metabolism at high temperature. J Exp Bot 59(7):1789–1798
Ho MY, Shen G, Canniffe DP, Zhao C, Bryant DA (2016) Light-dependent chlorophyll f synthase is a highly divergent paralog of PsbA of photosystem II. Science 353:aff9178
Hossain AS, Skalicky M, BresticM MS, Ashraful Alam M, Syed MA, Hossain J, Sarkar S, Saha S, Bhadra P et al (2021) Consequences and mitigation strategies of abiotic stresses in wheat ( Triticum aestivum L.) under the changing climate. Agronomy 11:241. https://doi.org/10.3390/agronomy11020241
Article CAS Google Scholar
Hsin J, Chandler DE, Gumbart J, Harrison CB, Şener M, Strumpfer J, Schulten K (2010) Self-assembly of photosynthetic membranes. ChemPhysChem 11(6):1154
Hsu, P. K., Takahashi, Y., Munemasa, S., Merilo, E., Laanemets, K., Waadt, R, Schroeder, J. I. (2018). Abscisic acid-independent stomatal CO 2 signal transduction pathway and convergence of CO2 and ABA signaling downstream of OST1 kinase.Pro Nat AcadSci 115(42) E9971-E9980.
Hu H, Boisson-Dernier A, Israelsson-Nordström M, Böhmer M, Xue S, Ries A, Schroeder JI (2010) Carbonic anhydrases are upstream regulators of CO 2 -controlled stomatal movements in guard cells. Nat Cell Biol 12(1):87–93
Hubbart S, Smillie IR, Heatley M, Swarup R, Foo CC, Zhao L, Murchie EH (2018) Enhanced thylakoid photoprotection can increase yield and canopy radiation use efficiency in rice. Commun Biol 1(1):1–12
Hussain S, Liu T, Iqbal N, Brestic M, Pang T, Mumtaz M, Li S, Wang L, Gao Y, Khan A (2020a) Effects of lignin, cellulose, hemicellulose, sucrose and monosaccharide carbohydrates on soybean physical stem strength and yield in intercropping. Photochem Photobiol Sci 19(4):462–472
Hussain S, Pang T, Iqbal N, Shafiq I, Skalicky M, Brestic M, Safdar ME, Mumtaz M, Ahmad A, Asghar MA, Raza A (2020b) Acclimation strategy and plasticity of different soybean genotypes in intercropping. Func Plant Biol 47(7):592–610
IPCC (2007) In: Solomon S, Qin D, Manning M, Chen Z, Marquis M, Averyt KB, Tignor M, Miller H.(eds.), Climate Change 2007: the physical science basis. Contribution of Working Group I to the Fourth Assessment Report of the Intergovernmental Panel on Climate Change. Cambridge University Press, Cambridge (996 pp)
Itzhak Kurek O, Kai Chang T, Bertain SM, Madrigal A, Liu L, Lassner MW, Zhu G (2007) Enhanced thermostability of Arabidopsis Rubisco activase improves photosynthesis and growth rates under moderate heat stress. Plant Cell 19:3230–3241
Kaldenhoff R (2012) Mechanisms underlying CO 2 diffusion in leaves. Curr Opin Plant Biol 15:276–281
Kebeish R, Niessen M, Thiruveedhi K, Bari R, Hirsch HJ, Rosenkranz R, Stäbler N, Schönfeld B, Kreuzaler F, Peterhänsel C. 2007. Chloroplastic photorespiratory bypass increases photosynthesis and biomass production in Arabidopsis thaliana. Nat Biotechnol 25:593–599
Keenan TF, Hollinger DY, Bohrer G, Dragoni D, Munger JW, Schmid HP, Richardson AD (2013) Increase in forest water-use efficiency as atmospheric carbon dioxide concentrations rise. Nature 499(7458):324–327
Kirst H, Gabilly ST, Niyogi KK, Lemaux PG, Melis A (2017) Photosynthetic antenna engineering to improve crop yields. Planta 245:1009–1020
Köhler IH, Ruiz-Vera UM, VanLoocke A, Thomey ML, Clemente T, Long SP, Bernacchi CJ (2017) Expression of cyanobacterial FBP/SBPase in soybean prevents yield depression under future climate conditions. J Exp Bot 68(3):715–726
Kromdijk J, Głowacka K, Leonelli L, Gabilly ST, Iwai M, NiyogiKK LSP (2016) Improving photosynthesis and crop productivity by accelerating recovery from photoprotection. Science 354:857–861
Kubien DS, von Cammerer S, Furbank RT, Sage RF (2003) C4 photosynthesis at low temperature. A study using transgenic plants with reduced amounts of Rubisco. Plant Physiol 132:1577–1585
Kubis A, Bar-Even A (2019) Synthetic biology approaches for improving photosynthesis. J Exp Bot 70(5):1425–1433
Kumarathunge DP, Medlyn BE, Drake JE, Tjoelker MG, Aspinwall MJ, Battaglia M, Cano FJ, Carter KR, Cavaleri MA, Cernusak LA, Chambers JQ (2019) Acclimation and adaptation components of the temperature dependence of plant photosynthesis at the global scale. New Phytol 222(2):768–784
Kumari VV, Roy A, Vijayan R, Banerjee P, Verma VC, Nalia A, Pramanik M, Mukherjee B, Ghosh A, Reja MH et al (2021) Drought and Heat stress in cool-season food legumes in sub-tropical regions: consequences, adaptation, and mitigation strategies. Plants 10:1038. https://doi.org/10.3390/plants10061038
Kurek I, Chang TK, Bertain SM, Madrigal A, Liu L, Lassner MW, Zhu G (2007) Enhanced thermostability of Arabidopsis Rubisco activase improves photosynthesis and growth rates under moderate heat stress. Plant Cell 19(10):3230–3241
Lawson T, Vialet-Chabrand S (2019) Speedy stomata, photosynthesis and plant water use efficiency. New Phytol 221(1):93–98
Leakey ADB, Ainsworth EA, Bernacchi CJ, Rogers A, Long SP, Ort DR (2009) Elevated CO 2 effects on plant carbon, nitrogen, and water relations: six important lessons from FACE. J Exp Bot 60:2859–2876
Leister D (2012) How can the light reactions of photosynthesis be improved in plants? Front Plant Sci 3:199
Li X, Kristiansen K, Rosenqvist E, Liu F (2019) Elevated CO 2 modulates the effects of drought and heat stress on plant water relations and grain yield in wheat. J Agron Crop Sci 205:362–371
Lin MT, Occhialini A, Andralojc PJ, Parry MAJ, Hanson MR (2014) Nature 513:547–550
Liu J, Lu Y, Hua W, Last RL (2019) A new light on photosystem II maintenance in oxygenic photosynthesis. Front Plant Sci 10:975
Long SP, Ainsworth EA, Rogers A, Ort DR (2004) Rising atmospheric carbon dioxide: plants face the future. Annu Rev Plant Biol 55:591–628
Long SP, Marshall-Colon A, Zhu XG (2015) Meeting the global food demand of the future by engineering crop photosynthesis and yield potential. Cell 161(1):56–66
Long SP, Zhu XG, Naidu SL, Ort DR (2006) Can improvement in photosynthesis increase crop yields? Plant Cell Environ 29(3):315–330
López-Calcagno PE, Abuzaid AO, Lawson T, Raines CA (2017) Arabidopsis CP12 mutants have reduced levels of phosphoribulokinase and impaired function of the Calvin–Benson cycle. J Exp Bot 68:2285–2298
Lundgren MR, Mathers A, Baillie AL et al (2019) Mesophyll porosity is modulated by the presence of functional stomata. Nat Commun 10:2825
Lundgren MR, Fleming AJ (2020) Cellular perspectives for improving mesophyll conductance. The Plant J 101(4):845–857
Makino A, Mae T (1999) Photosynthesis and plant growth at elevated levels of CO2. Plant Cell Physiol 40(10):999–1006
Mali SC, Raj S, Trivedi R (2020) Nanotechnology a novel approach to enhance crop productivity. Biochem Biophys Rep 24:100821
McAusland L, VialetChabrand S, Jauregui I, Burridge A, HubbartEdwards S, Fryer MJ, Fryer MJ, King IP, King J, Pyke K, Edwards KJ, Carmo‐Silva E (2020) Variation in key leaf photosynthetic traits across wheat wild relatives is accession dependent not species dependent. New Phytol. https://doi.org/10.1111/nph.16832
McGrath JM, Long SP (2014) Can the cyanobacterial carbon-concentrating mechanism increase photosynthesis in crop species? A theoretical analysis. Plant Physiol 164:2247–2261
Momayyezi M, McKown AD, Bell SC, Guy RD (2020) Emerging roles for carbonic anhydrase in mesophyll conductance and photosynthesis. The Plant J 101(4):831–844
Moses T (2019) Shedding light on the power of light. Plant Physiol 179:775–777
Muñoz P, Munné-Bosch S (2018) Photo-oxidative stress during leaf, flower and fruit development. Plant Physiol 176(2):1004–1014
Murchie EH, Niyogi KK (2011) Manipulation of photoprotection to improve plant photosynthesis. Plant Physiol 155:86–92
Murchie EH, Ruban AV (2020) Dynamic non-photochemical quenching in plants: from molecular mechanism to productivity. Plant J 101(4):885–896
Murchie EH, Pinto M, Horton P (2009) Agriculture and the new challenges for photosynthesis research. New Phytol 181:532–552
Nelson N, Yocum CF (2006) Structure and function of photosystems I and II. Annu Rev Plant Biol 57:521–565
Nickelsen J, Rengstl B (2013) Photosystem II assembly: from cyanobacteria to plants. Ann Rev Plant Biol 64:609–635
Nowicka B (2019) Target genes for plant productivity improvement. J Biotechnol 298:21–34
Nowicka B, Ciura J, Szymańska R, Kruk J (2018) Improving photosynthesis, plant productivity and abiotic stress tolerance – current trends and future perspectives. J Plant Physiol 231:415–433
Nuccio ML, Wu J, Mowers R, Zhou HP, Meghji M, Primavesi LF, Paul MJ, Chen X, Gao Y, Haque E, Basu SS (2015) Expression of trehalose-6-phosphate phosphatase in maize ears improves yield in well-watered and drought conditions. Nat Biotechnol 33(8):862–869
Orr DJ, Pereira AM, da Fonseca-Pereira P, Pereira-Lima ÍA, Zsögön A, Araújo WL (2017) Engineering photosynthesis: progress and perspectives. F1000Research 6:1891
Ort DR, Melis A (2011) Optimizing antenna size to maximize photosynthetic efficiency. Plant Physiol 155:79–85
Ort DR, Merchant SS, Alric J et al (2015) Redesigning photosynthesis to sustainably meet global food and bioenergy demand. Proc Nat Acad Sci 112:8529–8536
Parry MA, Keys AJ, Madgwick PJ, Carmo-Silva AE, Andralojc PJ (2008) Rubisco regulation: a role for inhibitors. J Exp Bot 59(7):1569–1580
Pearce FG (2006) Catalytic by-product formation and ligand binding by ribulose bisphosphate carboxylases from different phylogenies. Biochem J 399(3):525–534
Prashar A, Yildiz J, McNicol JW, Bryan GJ, Jones HG (2013) Infra-red thermography for high throughput field phenotyping in Solanumtuberosum. PLoS ONE 8(6):e65816
Price GD, Pengelly JJ, Forster B, Du J, Whitney SM, von Caemmerer S, Badger MR, Howitt SM, Evans JR (2013) The cyanobacterial CCM as a source of genes for improving photosynthetic CO 2 fixation in crop species. J Exp Bot 64:753–768
Raven JA (2013) R ubisco: still the most abundant protein of Earth? New Phytol 198(1):1–3
Ren T, Weraduwage SM, Sharkey TD (2019) Prospects for enhancing leaf photosynthetic capacity by manipulating mesophyll cell morphology. J Exp Bot 70(4):1153–1165
Robson TM, Klem K, Urban O, Jansen MAK (2015) Re-interpreting plant morphological responses to UV-B radiation. Plant Cell Environ 38:856–866
Ruban AV (2016) Non photochemical chlorophyll fluorescence quenching: mechanism and effectiveness in protecting plants from photodamage. Plant Physiol 170(4):1903–1916
Ruiz-Vera UM, Siebers M, Gray SB, Drag DW, Rosenthal DM, Kimball BA (2013) Global warming can negate the expected CO 2 stimulation in photosynthesis and productivity for soybean grown in the Midwestern United States. Plant Physiol 162(1):410–423
Ruiz-Vera UM, De Souza AP, Ament MR, Gleadow RM, Ort DR (2021) High sink strength prevents photosynthetic down-regulation in cassava grown at elevated CO 2 concentration. J Exp Bot 72(2):542–560
Sage RF, Kubien DS (2007) The temperature response of C3 and C4 photosynthesis. Plant Cell Environ 30:1086–1106
Salvucci ME, Crafts-Brandner SJ, Salvucci ME (2004) Relationship between the heat tolerance of photosynthesis and the thermal stability of rubisco activase in plants from contrasting thermal environments. Plant Physiol 134:1460–1470
Savir Y, Noor E, Milo R, Tlusty T (2010) Cross-species analysis traces adaptation of Rubisco toward optimality in a low-dimensional landscape. Proc Nat Acad Sci 107(8):3475–3480
Sawchuk MG, Donner TJ, Head P, Scarpella E (2008) Unique and overlapping expression patterns among members of photosynthesis-associated nuclear gene families in Arabidopsis. Plant Physiol 148(4):1908–1924
Sedelnikova OV, Hughes TE, Langdale JA (2018) Understanding the genetic basis of C4 Kranz anatomy with a view to engineering C3 crops. Ann Rev Gene 52:249–270
Seneweera S (2011) Effects of elevated CO 2 on plant growth and nutrient partitioning of rice ( Oryza sativa L.) at rapid tillering and physiological maturity. J Plant Interact 6:35–42
Serbin SP, Dillaway DN, Kruger EL, Townsend PA (2012) Leaf optical properties reflect variation in photosynthetic metabolism and its sensitivity to temperature. JExpBot 63(1):489–502
Sharwood RE (2017) Engineering chloroplasts to improve Rubisco catalysis: prospects for translating improvements into food and fiber crops. New Phytol 213:494–510
Shen BR, Wang LM, Lin XL et al (2019) Engineering a new chloroplastic photorespiratory bypass to increase photosynthetic efficiency and productivity in rice. Mol Plant 12:199–214
Silva-Pérez V, De Faveri J, Molero G, Deery DM, Condon AG, Reynolds MP, Evans JR, Furbank RT (2020) Genetic variation for photosynthetic capacity and efficiency in spring wheat. J Exp Bot 717:2299–2311. https://doi.org/10.1093/jxb/erz439
Simkin AJ, Lopez-Calcagno PE, Davey PA, Headland LR, Lawson T, Timm S, Bauwe H, Raines CA (2017a) Simultaneous stimulation of sedoheptulose 1,7-bisphosphatase, fructose 1,6-bisphophate aldolase and the photorespiratory glycine decarboxylase-H protein increases CO2 assimilation, vegetative biomass and seed yield in Arabidopsis. Plant Biotechnol J15:805–816
Simkin AJ, McAusland L, Headland LR, Lawson T, Raines CA (2015) Multigene manipulation of photosynthetic carbon assimilation increasesCO2 fixation and biomass yield in tobacco. J Bot 66:4075–4090
Simkin AJ, McAusland L, Lawson T, Raines CA (2017b) Overexpression of the Rieske FeS protein increases electron transport rates and biomass yield. Plant Physiol 175:134–145
Simkin AJ, López-Calcagno PE, Raines CA (2019) Feeding the world: improving photosynthetic efficiency for sustainable crop production. J Exp Bot 70(4):1119–1140
Sinclair TR, Jamieson PD (2006) Grain number, wheat yield, and bottling beer: an analysis. Field Crop Res 98:60–67
Sinclair TR, Jamieson PD (2008) Yield and grain number of wheat: a correlation or causal relationship? Authors’ response to the ‘“The importance of grain or kernel number in wheat: a reply to Sinclair and Jamieson”’ by R.A. Fischer Field Crop Res 105:22–26
Sinclair TR, Rufty TW, Lewis RS (2019) Increasing photosynthesis: unlikely solution for world food problem. Trends Plant Sci 24(11):1032–1039
Slattery RA, Walker BJ, Weber APM, Ort DR (2018) The impacts of fluctuating light on crop performance. Plant Physiol 176:990–1003
Smith NG, Keenan TF, Colin Prentice I, Wang H, Wright IJ, Niinemets U (2019) Global photosynthetic capacity is optimized to the environment. Ecol Lett 22(3):506–517
Song Q-F, Wang Y, Qu M, Ort DR, Zhu X-G (2017) The impact of modifying photosystem antenna size on canopy photosynthetic efficiency. Plant Cell Environ 40:2946–2957
South PF, Cavanagh AP, Liu HW, Ort DR (2019) Synthetic glycolate metabolism pathways stimulate crop growth and productivity in the field. Science 363:eaat077
South PF, Walker BJ, Cavanagh AP (2017) Bile Acid Sodium Symporter BASS6 Can Transport Glycolate and Is Involved in Photorespiratory Metabolism in Arabidopsis thaliana . Plant Cell 29(4):808–823
Spreitzer RJ (2003) Role of the small subunit in ribulose-1, 5-bisphosphate carboxylase/oxygenase. Arch Biochem Biophy 414(2):141–149
Srinivasan V, Kumar P, Long SP (2017) Decreasing, not increasing, leaf area will raise crop yields under global atmospheric change. Glob Chang Biol 23:1626–1635
Takizawa K, Kanazawa A, Kramer DM (2008) Depletion of stromal P(i) induces high ‘energy-dependent’ antenna exciton quenching (q(E)) by decreasing proton conductivity at CF(O)-CF(1) ATP synthase. Plant Cell Environ 31:235–243
Tausz M, Tausz-Posch S, Norton RM, Fitzgerald GJ, Nicolas ME, Seneweera S (2013) Understanding crop physiology to select breeding targets and improve crop management under increasing atmospheric CO 2 concentrations. Environ Exp Bot 88:71–80
Taylor SH, Long SP (2017) Slow induction of photosynthesis on shade tosunto sun transitions in wheat may cost at least 21% of productivity. Philos Trans R Soc B 372:20160543
Tcherkez G (2013) Modelling the reaction mechanism of ribulose-1, 5-bisphosphate carboxylase/oxygenase and consequences for kinetic parameters. Plant Cell Environ 36(9):1586–1596
Tester M, Langridge P (2010) Breeding technologies to increase crop production in a changing world. Science 327:818–822
Thompson M, Gamage D, Hirotsu N, Martin A, Seneweera S (2017) Effects of elevated carbon dioxide on photosynthesis and carbon partitioning: a perspective on root sugar sensing and hormonal crosstalk. Front Physiol 8:578
Timm S, Giese J, Engel N, Wittmiß M, Florian A, Fernie AR, Bauwe H (2018) T-protein is present in large excess over the other proteins of the glycine cleavage system in leaves of Arabidopsis. Planta 247:41–51
Ubierna N, Gandin A, Cousins AB (2018) The response of mesophyll conductance to short-term variation in CO2 in the C4 plants Setariaviridis and Zea mays. J Exp Bot 69(5):1159–1170. https://doi.org/10.1093/jxb/erx464
Vandegeer R, Miller R, Bain M (2012) Drought adversely affects tuber development and nutritional quality of the staple crop cassava (Manihot esulenta Crantz). Funct Plant Biol 40:195–200
Von Caemmerer S, Quick WP, Furbank RT. (2012). The development ofC4 rice: current progress and future challenges. Science 336, 1671–1672.
von Caemmerer S, Evans JR (2010) Enhancing C3 photosynthesis. Plant Physiol 154(2):589–592
Wachter, R.M. and Henderson, J.N., 2015. Photosynthesis: rubisco rescue. Nat Plants 1(1), pp.1–2.
Walker BJ, Drewry DT, Slattery RA, VanLoocke A, Cho YB, Ort DR (2018) Chlorophyll can be reduced in crop canopies with little penalty to photosynthesis. Plant Physiol 176(2):1215–1232
Wallace JG, Rodgers-Melnick E, Buckler ES (2018) On the road to breeding 4.0: unraveling the good, the bad, and the boring of crop quantitative genomics. Ann Rev Gene. 52:421–444
Wang LM, Shen BR, Li BD, Zhang CL, Lin M, Tong PP, Peng XX (2020) A synthetic photorespiratory shortcut enhances photosynthesis to boost biomass and grain yield in rice. Mol Plant 13(12):1802–1815
Wang P, Hendron RW, Kelly S (2017) Transcriptional control of photosynthetic capacity: conservation and divergence from Arabidopsis to rice. New Phytol 216:32–45
Wang QL, Chen JH, He NY, Guo FQ (2018) Metabolic reprogramming in chloroplasts under heat stress in plants. Int J Mol Sci 19(3):849
PubMed Central Google Scholar
Way DA, Yamori W (2014) Thermal acclimation of photosynthesis: on the importance of adjusting our definitions and accounting for thermal acclimation of respiration. Photosynth Res 119(1–2):89–100
Wise RR, Olson AJ, Schrader SM, Sharkey TD (2004) Electron transport is the functional limitation of photosynthesis in field-grown Pima cotton plants at high temperature. Plant Cell Environ 27:717–724
Wu A, Hammer GL, Doherty A, von Caemmerer S, Farquhar GD (2019) Quantifying impacts of enhancing photosynthesis on crop yield. Nat Plants 5(4):380–388
Xin CP, Tholen D, Devloo V, Zhu XG (2015) The benefits of photorespiratory bypasses: how can they work? Plant Physiol 167:574–585
Yadav SK, Khatri K, Rathore MS, Jha B (2018) Introgression of UfCyt c6, a thylakoid lumen protein from a green seaweed Ulva fasciata Delile enhanced photosynthesis and growth in tobacco. Mol Biol Rep 45:1745–1758
Yamori W, Von Caemmerer S (2009) Effect of Rubisco activase deficiency on the temperature response of CO2 assimilation rate and Rubisco activation state: insights from transgenic tobacco with reduced amounts of Rubisco activase. Plant Physiol 151:2073–2082
Yamori W, Noguchi K, Hikosaka K, Terashima I (2010) Phenotypic plasticity in photosynthetic temperature acclimation among crop species with different cold tolerances. Plant Physiol 152:388–399
Yamori W, Hikosaka K, Way DA (2014) Temperature response of photosynthesis in C3, C4, and CAM plants: temperature acclimation and temperature adaptation. Photosynth Res 119:101–117
Yoon M, Putterill JJ, Ross GS, Laing WA (2001) Determination of the relative expression levels of Rubisco small subunit genes in Arabidopsis by rapid amplification of cDNA ends. Ana Biochem 291(2):237–244
Zhang J et al (2018) Insights into the molecular mechanisms of CO 2 -mediated regulation of stomatal movements. Curr Biol 28:R1356–R1363
Zheng Y, Li F, Hao L, Yu J, Guo L, Zhou H, Ma C, Zhang X, Xu M (2019) Elevated CO 2 concentration induces photosynthetic down-regulation with changes in leaf structure, non-structural carbohydrates and nitrogen content of soybean. BMC Plant Biol 19(1):1–18
Zhou XT, Wang F, Ma YP, Jia LJ, Liu N, Wang HY, Zhao P, Xia GX, Zhong NQ (2018) Ectopic expression of SsPETE2, a plastocyanin from Suaedasalsa, improves plant tolerance to oxidative stress. Plant Sci 268:1–10
Zhu XG, de Sturler E, Long SP (2007) Optimizing the distribution of resources between enzymes of carbon metabolism can dramatically increase photosynthetic rate: a numerical simulation using an evolutionary algorithm. Plant Physiol 145:513–526
Zhu X-G, Long SP, Ort DR (2010) Improving photosynthetic 1534 efficiency for greater yield. Ann Rev Plant Biol 61:235–261
Zhu XG, Portis AR Jr, Long SP (2004) Would transformation of C3 crop plants with foreign Rubisco increase productivity? A computational analysis extrapolating from kinetic properties to canopy photosynthesis. Plant Cell Environ 27(2):155–165
Ziotti ABS, Silva BP, Neto MC (2019) Photorespiration is crucial for salinity acclimation in castor bean. Environ Exp Bot. https://doi.org/10.1016/j.envexpbot.2019.103845
Download references
Acknowledgements
The research was supported by the National Natural Science Foundation of China (31871570) and Sichuan Innovation Team Project of National Modern Agricultural Industry Technology System (SCCXTD-2020-20) and VEGA-1-0589-19 & APVV-18-0465. And Collaborative Innovation Center for Modern Crop Production co-sponsored by Province and Ministry (CIC-MCP), the Science and Technology Department of Zhejiang Province (14th 5-year New Oil Crops Breeding).
Author information
Sajad Hussain and Zaid Ulhassan have contributed equally to this work.
Authors and Affiliations
College of Agronomy, Sichuan Agricultural University, 211-Huimin Road, Wenjiang District, Chengdu, 611130, People’s Republic of China
Sajad Hussain, Wenyu Yang & Weiguo Liu
Key Laboratory of Crop Ecophysiology and Farming System in Southwest China (Ministry of Agriculture), Sichuan Engineering Research Center for Crop Strip Intercropping System, Sichuan Agricultural University, Chengdu, People’s Republic of China
Institute of Crop Science, Ministry of Agriculture and Rural Affairs Laboratory of Spectroscopy Sensing, Zhejiang University, Hangzhou, 310058, People’s Republic of China
Zaid Ulhassan & Weijun Zhou
Department of Plant Physiology, Slovak University of Agriculture, 94976, Nitra, Slovakia
Marian Brestic & Marek Zivcak
К.A. Timiryazev Institute of Plant Physiology, Russian Academy of Sciences, Botanicheskaya St. 35, Moscow, Russia, 127276
Suleyman I. Allakhverdiev
Department of Plant Physiology, College of Life Sciences, Shandong Agricultural University, Daizong Road No. 61, 271018, Taian, People’s Republic of China
Xinghong Yang
College of Agriculture, University of Sargodha, Sargodha, Punjab, 40100, Pakistan
Muhammad Ehsan Safdar
You can also search for this author in PubMed Google Scholar
Corresponding authors
Correspondence to Wenyu Yang or Weiguo Liu .
Ethics declarations
Conflict of interest.
All authors declared no conflict of interest.
Additional information
Publisher's note.
Springer Nature remains neutral with regard to jurisdictional claims in published maps and institutional affiliations.
Rights and permissions
Reprints and permissions
About this article
Hussain, S., Ulhassan, Z., Brestic, M. et al. Photosynthesis research under climate change. Photosynth Res 150 , 5–19 (2021). https://doi.org/10.1007/s11120-021-00861-z
Download citation
Received : 27 February 2021
Accepted : 28 June 2021
Published : 07 July 2021
Issue Date : December 2021
DOI : https://doi.org/10.1007/s11120-021-00861-z
Share this article
Anyone you share the following link with will be able to read this content:
Sorry, a shareable link is not currently available for this article.
Provided by the Springer Nature SharedIt content-sharing initiative
- Photosynthesis
- Photosystem
- Assimilates
- Find a journal
- Publish with us
- Track your research

An official website of the United States government
The .gov means it’s official. Federal government websites often end in .gov or .mil. Before sharing sensitive information, make sure you’re on a federal government site.
The site is secure. The https:// ensures that you are connecting to the official website and that any information you provide is encrypted and transmitted securely.
- Publications
- Account settings
Preview improvements coming to the PMC website in October 2024. Learn More or Try it out now .
- Advanced Search
- Journal List
- Plant Cell Physiol

Photosynthetic Research in Plant Science
Ayumi tanaka.
1 Institute of Low Temperature Science, Hokkaido University, Sapporo, Japan
Amane Makino
2 Graduate School of Agricultural Science, Tohoku University, Sendai, Japan
The online version of this article has been published under an open access model. Users are entitled to use, reproduce, disseminate, or display the open access version of this article for non-commercial purposes provided that: the original authorship is properly and fully attributed; the Journal and the Japanese Society of Plant Physiologists are attributed as the original place of publication with the correct citation details given; if an article is subsequently reproduced or disseminated not in its entirety but only in part or as a derivative work this must be clearly indicated. For commercial re-use, please contact [email protected]
Photosynthesis is a highly regulated, multistep process. It encompasses the harvest of solar energy, transfer of excitation energy, energy conversion, electron transfer from water to NADP + , ATP generation and a series of enzymatic reactions that assimilate carbon dioxide and synthesize carbohydrate.
Photosynthesis has a unique place in the history of plant science, as its central concepts were established by the middle of the last century, and the detailed mechanisms have since been elucidated. For example, measurements of photosynthetic efficiency (quantum yield) at different wavelengths of light (Emerson and Lews 1943 ) led to the insight that two distinct forms of Chl must be excited in oxygenic photosynthesis. These results suggested the concept of two photochemical systems. The reaction center pigments of PSII and PSI (P680 and P700, respectively) were found by studying changes in light absorbance in the red region (Kok 1959 , Döring et al. 1969 ). Chls with absorbance maxima corresponding to these specific wavelengths were proposed as the final light sink. These Chls were shown to drive electron transfer by charge separation. The linkage of electron transfer and CO 2 assimilation was suggested by studies on Hill oxidant (Hill 1937 ). A linear electron transport system with two light-driven reactions (Z scheme) was proposed based upon observations of the redox state of cytochromes (Hill and Bendall 1960 , Duysens et al. 1961 ), and photophosphorylation was found to be associated with thylakoid fragments (Arnon et al. 1954 ). The metabolic pathway that assimilates carbon by fixation of CO 2 was discovered by Calvin's group who used 14 CO 2 radioactive tracers in the 1950s (Bassham and Calvin 1957 ). This was the first significant discovery in biochemistry made using radioactive tracers. The primary reaction of CO 2 fixation is catalyzed by Rubisco (Weissbach et al. 1956 ), initially called Fraction 1 protein (Wildman and Bonner 1947 ). Rubisco is the most abundant protein in the world, largely because it is also the most inefficient with the lowest catalytic turnover rate (1–3 s –1 ). Another CO 2 fixation pathway was then found in sugarcane (Kortschak et al. 1964, Hatch and Slack 1965) and named C 4 photosynthesis.
Although photosynthesis plays the central role in the energy metabolism of plants, historically there have not been strong interactions between photosynthesis research and other fields of plant science. Many techniques and tools developed for photosynthesis research have not been widely used in other fields because they were developed to examine phenomena unique to photosynthesis. For example, excitation energy transfer and charge separation are fundamental but unique processes of photosynthesis. Another reason for the historic isolation of photosynthesis research within plant science is that it was long believed that CO 2 fixation and carbohydrate production are the sole function of photosynthesis, with carbohydrates representing the only link between photosynthesis and other biological phenomena.
However, this situation has begun to change. Recent research has revealed that photosynthesis is closely related to a variety of other physiological processes. It is a major system for controlling the redox state of cells, playing an important role in regulating enzyme activity and many other cellular processes (Buchanan and Balmer 2005 , Hisabori et al. 2007 ). Photosynthesis also generates reactive oxygen species, which are now appreciated as being regulatory factors for many biological processes rather than inevitable by-products of photosynthesis (Wagner et al. 2004 , Beck 2005 ). Precursor molecules of Chl, which are a major component of photosynthesis, act as a chloroplast-derived signal, and are involved in regulating the cell cycle (Kobayashi et al. 2009 ). In light of this new information, it seems important to re-evaluate the function(s), both potential and demonstrated, of photosynthesis from a variety of view points. Photosynthesis research now employs the methods and tools of molecular biology and genetics, which are central methods for plant science in general. Meanwhile, Chl fluorescence and gas exchange measurements, developed especially for photosynthesis research, are now widely used in stress biology and ecology.
Photosynthesis research also contributes to our understanding of ecological phenomena and even the global environments (Farquhar et al. 1980 , de Pury and Farquhar 1997 , Monsi and Saeki 2005 ). Indeed, photosynthesis is now an integral component of simulation models used to predict the future of our planet. Improving the efficiency of photosynthesis by artificial modification of photosynthetic proteins and pathways has long been considered impossible or at best problematic, because, over evolutionary time, photosynthesis has become complex and tightly regulated. However, recent advances have made it possible to manipulate photosynthesis using molecular genetic technology (Andrews and Whiney 2003 , Raines 2006 ). These advances may have positive influences on crop productivity (Parry et al. 2007 ) as photosynthetic rates have frequently been correlated with biomass accretion (Kruger and Volin 2006 ). Thus, we can expect many more novel concepts to be added to this history of photosynthetic research.
As photosynthesis research tackles new challenges, we should also continue to re-evaluate past research. Oxygen evolution, energy dissipation and cyclic electron transport are crucial processes during photosynthesis, yet their mechanisms still remain to be clarified. We have very limited knowledge of the formation and degradation of photosynthetic apparatus. Also, although photosynthesis plays a central role in C and N metabolism in plants, we do not yet understand how potential photosynthesis is related to crop productivity.
Plant and Cell Physiology would like to contribute to the development of novel concepts, pioneering new fields and solving the unanswered questions of photosynthesis. This special issue covers a wide range of topics in photosynthesis research. Terashima et al. (pp. 684–697) readdress the enigmatic question of why leaves are green. They show that the light profile through a leaf is steeper than that of photosynthesis, and that the green wavelengths in white light are more effective in driving photosynthesis than red light. Evans (pp. 698–706) proposes a new model using Chl fluorescence to explore modifications in quantum yield with leaf depth. This new multilayered model can be applied to study variations in light absorption profiles, photosynthetic capacity and calculation of chloroplastic CO 2 concentration at different depths through the leaf.
Singlet oxygen, 1 O 2 , is produced by the photosystem and Chl pigments. 1 O 2 not only causes physiological damage but also activates stress response programs. The flu mutant of Arabidopsis thaliana overaccumulates protochlorophyllide that upon illumination generates singlet oxygen, causing growth cessation and cell death. Coll et al. (pp. 707–718) have isolated suppressor mutants, dubbed ‘singlet oxygen-linked death activator’ (soldat), that specifically abrogate 1 O 2 -mediated stress responses in young flu seedlings, and they discuss the processes of acclimation to stresses. Phephorbide a is a degradation product of Chl and one of the most powerful photosensitzing molecules. Mutants defective in pheophorbide a oxygenase, which converts phephorbide a to open tetrapyrrole, accumulate pheophorbide a and display cell death in a light-dependent manner. Hirashima et al. (pp. 719–729) report that pheophorbide a is involved in this light-independent cell death.
Plants regulate the redox level of the plastoquinone pool in response to the light environment. In acclimation to high-light conditions, the redox level is kept in an oxidized state by the plastoquinone oxidation system (POS). Miyake et al. (pp. 730–743) investigated the mechanism of POS using the Chl fluorescence parameter, qL.
Nagai and Makino (pp. 744–755) examine in detail the differences between rice and wheat, the two most commercially important crops, in the temperature responses of CO 2 assimilation and plant growth. They find that the difference in biomass production between the two species at the level of the whole plant depends on the difference in N-use efficiency in leaf photosynthesis and growth rate. Sage and Sage (pp. 756–772) examine chlorenchyma structure in rice and related Oryza species in relation to photosynthetic function. They find that rice chlorenchyma architecture includes adaptations to maximize the scavenging of photorespired CO 2 and to enhance the diffusive conductance of CO 2 . In addition, they consider that the introduction of Kranz anatomy does not require radical anatomical alterations in engineering C 4 rice.
Bioinformatics has become a powerful tool, especially in photosynthetic research, because photosynthetic organisms have a wide taxonomic distribution among prokaryotes and eukaryotes. Ishikawa et al. (pp. 773–788) present the results of a pilot study of functional orthogenomics, combining bioinformatic and experimental analyses to identify nuclear-encoded chloroplast proteins of endosymbiontic origin (CRENDOs). They conclude that phylogenetic profiling is useful in finding CPRENDOs, although the physiological functions of orthologous genes may be different in chloroplasts and cyanobacteria.
We hope you enjoy this special issue, and would like to invite you to submit more excellent papers to Plant and Cell Physiology in the field of photosynthesis.
- Andrews TJ, Whitney SM. Manipulating ribulose bisphosphate carboxylase/oxygenase in the chloroplasts of higher plants. Arch. Biochem. Biophys. 2003; 414 :159–169. [ PubMed ] [ Google Scholar ]
- Arnon DI, Allen MB, Whatley FR. Photosynthesis by isolated chloroplasts. Nature. 1954; 174 :394–396. [ PubMed ] [ Google Scholar ]
- Bassham JA, Calvin M. The Path of Carbon in Photosynthesis. New Jersey: Prentice-Hall; 1957. [ Google Scholar ]
- Beck CF. Signaling pathways from the chloroplast to the nucleus. Planta. 2005; 222 :743–756. [ PubMed ] [ Google Scholar ]
- Buchanan BB, Balmer Y. Redox regulation: a broadening horizon. Annu. Rev. Plant Biol. 2005; 56 :187–220. [ PubMed ] [ Google Scholar ]
- Coll NS, Danon A, Meurer J, Cho WK, Apel K. Characterization of soldat8 , a suppressor of singlet oxygen-induced cell death in Arabidopsis seedlings. Plant Cell Physiol. 2009; 50 :707–718. [ PubMed ] [ Google Scholar ]
- de Pury DGG, Farquhar GD. Simple scalling of photo-synthesis from leaves to canopies without the errors of big-leaf models. Plant Cell Environ. 1997; 20 :537–557. [ Google Scholar ]
- Döring G, Renger G, Vater J, Witt HT. Properties of the photoactive chlorophyll-aII in photosynthesis. Z. Naturforsch. B. 1969; 24 :1139–1143. [ PubMed ] [ Google Scholar ]
- Duysens JNM, Amesz J, Kamp BM. Two photochemical systems in photosynthesis. Nature. 1961; 190 :510–511. [ PubMed ] [ Google Scholar ]
- Emerson R, Lews ML. The dependence of the quantum yield of chlorella photosynthesis on wave length of light. Amer. J. Bot. 1943; 30 :165–178. [ Google Scholar ]
- Evans JR. Exploring chlorophyll fluorescence with a multilayer leaf model. Plant Cell Physiol. 2009; 50 :698–706. [ PubMed ] [ Google Scholar ]
- Farquhar GD, von Caemmerer S, Berry JA. A biochemical model of photosynthetic CO 2 assimilation in leaves of C 3 species. Planta. 1980; 149 :78–90. [ PubMed ] [ Google Scholar ]
- Hatch MD, Slack CR. Photosynthesis by sugarcane leaves. A new carboxylation reaction and the pathway of sugar formation. Biochem. J. 1966; 101 :103–111. [ PMC free article ] [ PubMed ] [ Google Scholar ]
- Hill R. Oxygen evolved by isolated chloroplasts. Nature. 1937; 139 :881–882. [ Google Scholar ]
- Hill R, Bendall F. Function of the two cytochrome components in chloroplasts: a working hypothesis. Nature. 1960; 186 :136–137. [ Google Scholar ]
- Hirashima M, Tanaka R, Tanaka A. Light-independent cell death induced by accumulation of pheophorbide a in Arabidopsis thaliana . Plant Cell Physiol. 2009; 50 :719–729. [ PubMed ] [ Google Scholar ]
- Hisabori T, Motohashi K, Hosoya-Matsuda N, Ueoka-Nakanishi H, Romano PG. Towards a functional dissection of thioredoxin networks in plant cells. Photochem. Photobiol. 2007; 83 :145–151. [ PubMed ] [ Google Scholar ]
- Ishikawa M, Fujiwara M, Sonoike K, Sato N. Orthogeno-mics of photosynthetic organisms: bioinformatic and experimental analysis of chloroplast proteins of endosymbiotic origin in Arabidopsis and their counterparts in Synechocystis . Plant Cell Physiol. 2009; 50 :773–788. [ PubMed ] [ Google Scholar ]
- Kobayashi Y, Kanesaki Y, Tanaka A, Kuroiwa H, Kuroiwa T, Tanaka K. Tetrapyrrole signal as a cell-cycle coordinator from organelle to nuclear DNA replication in plant cells. Proc. Natl Acad. Sci. USA. 2009; 106 :803–807. [ PMC free article ] [ PubMed ] [ Google Scholar ]
- Kok B. Light induced absorption changes in photosynthetic organisms. II. A split-beam difference spectrophotometer. Plant Physiol. 1959; 34 :184–192. [ PMC free article ] [ PubMed ] [ Google Scholar ]
- Kortschak HP, Harrt CE, Burr GO. Carbon dioxide fixation in sugarcane leaves. Plant Physiol. 1965; 40 :209–213. [ PMC free article ] [ PubMed ] [ Google Scholar ]
- Kruger EL, Volin JC. Reexamining the empirical relation between plant growth and leaf photosynthesis. Funct. Plant Biol. 2006; 33 :421–429. [ PubMed ] [ Google Scholar ]
- Miyake C, Amako K, Shiraishi N, Sugimoto T. Acclimation of tobacco leaves to high light intensity drives the plastoquinone oxidation system—relationship among the fraction of open PSII centers, non-photochemical quenching of Chl fluorescence and the maximum quantum yield of PSII in the dark. Plant Cell Physiol. 2009; 50 :730–743. [ PubMed ] [ Google Scholar ]
- Monsi M, Saeki T. On the factor light in plant communities and its importance for matter production. (English version of Jpn. J. Bot . 14: 22–52, 1953). Ann. Bot. 2005; 95 :549–567. [ PMC free article ] [ PubMed ] [ Google Scholar ]
- Nagai T, Makino A. Differences between rice and wheat in temperature responses of photosynthesis and plant growth. Plant Cell Physiol. 2009; 50 :744–755. [ PMC free article ] [ PubMed ] [ Google Scholar ]
- Parry MAJ, Madgwick PJ, Carvalho JFC, Andralojc PJ. Prospects from increasing photosynthesis by overcoming the limitations of Rubisco. J. Agric. Sci. 2007; 145 :31–43. [ Google Scholar ]
- Raines CA. Transgenic approaches to manipulate the environmental responses of the C-3 carbon fixation cycle. Plant Cell Environ. 2006; 29 :331–339. [ PubMed ] [ Google Scholar ]
- Sage TL, Sage RF. The functional anatomy of rice leaves: implications for refixation of photorespiratory CO 2 and efforts to engineer C 4 photosynthesis into rice. Plant Cell Physiol. 2009; 50 :756–772. [ PubMed ] [ Google Scholar ]
- Terashima I, Fujita T, Inoue T, Chow WS, Oguchi R. Green light drives leaf photosynthesis more efficiently than red light in strong white light: revisiting the enigmatic question of why leaves are green. Plant Cell Physiol. 2009; 50 :684–697. [ PubMed ] [ Google Scholar ]
- Wagner D, Przybyla D, Op den Camp R, Kim C, Landgraf F, Lee KP, et al. The genetic basis of singlet oxygen-induced stress responses of Arabidopsis thaliana. Science. 2004; 306 :1183–1185. [ PubMed ] [ Google Scholar ]
- Weissbach A, Horecker BL, Hurwitz J. The enzymatic formation of phosphoglyceric acid from ribulose diphosphate and carbon dioxide. J. Biol. Chem. 1956; 218 :795–810. [ PubMed ] [ Google Scholar ]
- Wildman SG, Bonner J. The proteins of green leaves. I. Isolation, enzymic properties, and auxin contents of spinach cytoplasmic proteins. Arch. Biochem. 1947; 14 :381–413. [ PubMed ] [ Google Scholar ]
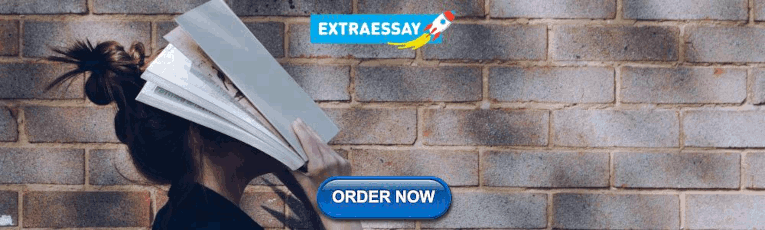
IMAGES
VIDEO
COMMENTS
Introduction. The photosynthetic activity of light is wavelength dependent. Based on McCree's work (McCree, 1971, 1972), photosynthetically active radiation is typically defined as light with a wavelength range from 400 to 700 nm.Light with a wavelength shorter than 400 nm or longer than 700 nm was considered as unimportant for photosynthesis, due to its low quantum yield of CO 2 ...
(a) Light-emitting diode light(s) can sustain normal plant growth. Pioneer experiments on plant growth under red LEDs on lettuce were reported by Bula et al.[]Martineau et al. [] calculated that the amounts of dry matter per mole of artificial lighting gained by lettuce grown using red (650 nm) LEDs or high-pressure sodium lamps were identical, and Chang et al.
Introduction. Photoinhibition often occurs when light energy is excessive, which reduces photochemical efficiency and even causes photooxidative system damage (Ma et al., 2015; Dias et al., 2018).Furthermore, low light intensity influences photosynthesis, which is central to plant productivity, and can therefore severely restrict plant growth (Zhu et al., 2014), and even death (Wang et al., 2021).
The impacts of wavelengths in 500-600 nm on plant response and their underlying mechanisms remain elusive and required further investigation. Here, we investigated the effect of light quality on leaf area growth, biomass, pigments content, and net photosynthetic rate (Pn) across three Arabidopsis thaliana accessions, along with changes in transcription, photosynthates content, and ...
Since 1893, when the word "photosynthesis" was first coined by Charles Reid Barnes and Conway MacMillan, our understanding of the elements and regulation of this complex process is far from being entirely understood. We aim to review the most relevant advances in photosynthesis research from the last few years and to provide a perspective ...
In light of the (expected) impact of thylakoid electron transport rate, the rate of regulation of the light reactions and the longevity of the photosynthetic activity over the growing season, I will focus in this review on approaches that aim to modify the light reactions of photosynthesis, i.e., on attempts to increase ε conversion (for an overview see Figure 1) and only refer in passing to ...
We attribute this feature to the ground-state bleaching of the photoactive reaction centres of PSI and PSII. A lower-energy feature at 715 nm grew within roughly 2 ps after photo-excitation and ...
1. Introduction. Photosynthesis is a complex metabolic process, in which the energy of light, captured by an elaborate system of pigment-containing proteins, is used for the reduction of CO 2, which is an essential step in the biosynthesis of organic compounds.As photosynthesis uses the most abundant energy source available on Earth, it is the most important biochemical process in biological ...
Search for more papers by this author , Parisa Heydarizadeh. Parisa Heydarizadeh ... Shed light on photosynthetic organisms: a physical perspective to correct light measurements, Photosynthesis Research, 10.1007/s11120-023-01001-5. ... effects on the organization of light-harvesting complex II and photosystem II functions, Photosynthesis ...
Herein, photosynthesis could be a blueprint for the design of efficient systems to capture sunlight and store it in chemicals (Nagy and Garab 2021). On the other hand, developing technology in plant research could improve light use efficiency and ultimately the performance of cultivated species, leading to increased food production.
How PhotoRedesign will address limitations of photosynthesis and attempt to enhance the process. (a) The overall maximal efficiency of the light reactions (in terms of the fraction of incident solar energy converted into chemical energy) is theoretically only 26% (Zhu et al., 2010).Taking into account carbon fixation, this value does normally not exceed ˜1% in crop plants under field ...
In this Perspective article, we describe the visions of the PhotoRedesign consortium funded by the European Research Council of how to enhance photosynthesis. The light reactions of photosynthesis in individual phototrophic species use only a fraction of the solar spectrum, and high light intensities can impair and even damage the process.
Photosynthesis Research is an international journal publishing research in all areas related to photosynthesis. Discusses both basic and applied aspects of photosynthesis. Welcomes research in all photosynthetic systems, including natural organisms and biomimetic systems. Includes research at all levels of plant organization: molecular ...
The protonation not only extends the light absorption of the COF but also provides proton sources that directly participate in H2O2 generation. Notably, the protonation simplifies the reaction pathways of ORR to H2O2, i.e. from an indirect superoxide radical ([[EQUATION]]) mediated route to a direct one-step two-electron route.
Photosynthetic Research in Plant Science. Photosynthesis is a highly regulated, multistep process. It encompasses the harvest of solar energy, transfer of excitation energy, energy conversion, electron transfer from water to NADP +, ATP generation and a series of enzymatic reactions that assimilate carbon dioxide and synthesize carbohydrate ...
On the simulation and interpretation of substrate-water exchange experiments in photosynthetic water oxidation. Petko Chernev. A. Orkun Aydin. Johannes Messinger. Research Open access 21 March 2024. Part of 1 collection: Special Issue: Energy Conversion Reactions in Natural and Artificial Photosynthesis.
A generalised dynamic model of leaf-level C3 photosynthesis combining light and dark reactions with stomatal behavior. Photosynthesis Research 141: 99-118. [Google Scholar] Belyaeva NE. 2004. Generalized model of primary photosynthetic processes in chloroplasts. PhD thesis, Lomonosov Moscow State University. [Google Scholar]
The process of. photosynthesis in plants is based on two reactions that are carried out by separate parts. of the chloroplast. The light reactions occur in the chloroplast thylakoid membrane and ...
The first idea of artificial photosynthesis dates back to the beginning of 1900s when Giacomo Ciamician commented in his paper that human should shift from consuming fossil fuels to generating sustainable energy from the sun. 2 The first experimental demonstration of light-driven water splitting was so called "Honda-Fujishima effect". 3 ...
Journal of Integrative Agriculture 2015, 14(9): 1755â€"1766 RESEARCH ARTICLE Available online at www.sciencedirect.com ScienceDirect Effects of light intensity on photosynthesis and photoprotective mechanisms in apple under progressive drought MA Ping, BAI Tuan-hui, WANG Xiao-qian, MA Feng-wang College of Horticulture, Northwest A&F University, State Key Laboratory of Crop Stress Biology ...
Abstract. Recent research has proposed to modify leaf-colour traits to improve canopy photosynthesis (A c) by allowing light penetration to lower layers of a dense canopy.Whether and how enhanced light penetration can really increase A c and whether leaf-colour modification influences other growth-related traits remain unclear. Canopy light and nitrogen profile parameters (i.e., the extinction ...
Photosynthesis may be split into the 'light' and 'dark' reactions. In the light reactions, water is split using light into oxygen, protons and electrons, and in the dark reactions, the protons and electrons are used to reduce CO 2 to carbohydrate (given here by the general formula CH 2 O). The two processes can be summarized thus:
A research team led by the Hong Kong University of Science and Technology (HKUST) has discovered how carboxysomes—carbon-fixing structures found in some bacteria and algae—work.
Photosynthesis is the main supplier of carbon and energy required for the synthesis of the organic compounds that drive plant growth and development (Simkin et al. 2019).In this way, increasing photosynthesis-related CO 2 assimilation per unit leaf/land area could be ideal to boost crop production. Over the coming decades, the increasing global population and climate change uncertainties will ...
Cotton photosynthetic efficiency and the root-shoot relationship are two important physiological indexes affecting the final yield, but the interactive effects of plastic film mulching and planting density on the cotton photosynthetic efficiency and root-shoot relationship have rarely been reported. We aimed to investigate the optimal plant density with or without plastic film mulching for ...
Introduction. Photosynthesis is a process that all life on earth depends on. Photosynthetic organisms convert more than 10 9 metric tons of atmospheric CO 2 into biomass per year. With the global human population rising from ~7 billion now to 9-10 billion by 2050, the worldwide trend towards a more meat-rich human diet, the loss of harvest and grazing land, and the negative effects of global ...
Photosynthetic Research in Plant Science. Photosynthesis is a highly regulated, multistep process. It encompasses the harvest of solar energy, transfer of excitation energy, energy conversion, electron transfer from water to NADP +, ATP generation and a series of enzymatic reactions that assimilate carbon dioxide and synthesize carbohydrate ...