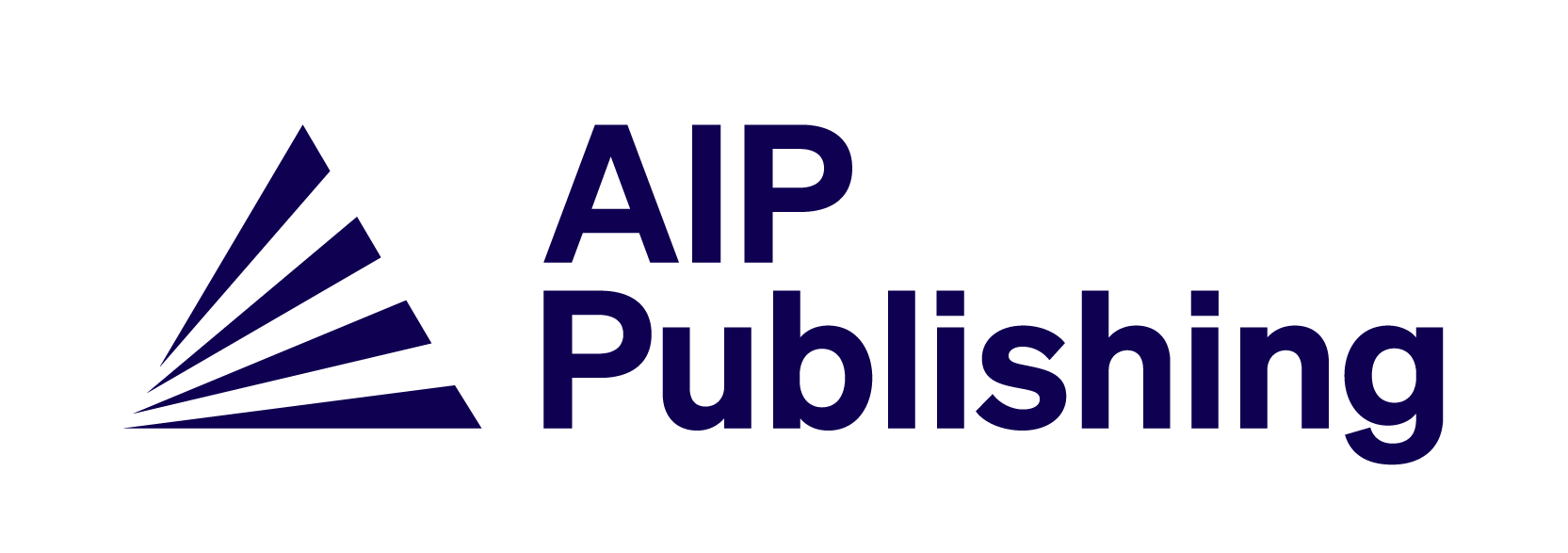
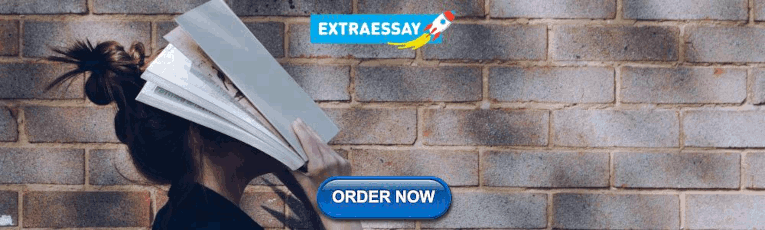
Focus and Coverage
The Journal of Chemical Physics is an international journal that publishes cutting edge research in all areas of modern physical chemistry and chemical physics. The Journal also publishes brief communications of significant new findings, perspectives on the latest advances in the field, and Special Topics.
Read more about the journal
Editor-in-Chief
Tianquan (Tim) Lian
Current Issue
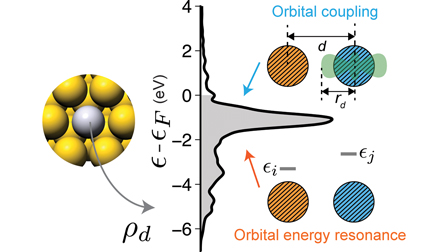
Submit your article
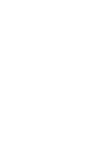
Sign up for alerts
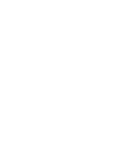
Active Topics
- Computational chemistry
- Electronic structure
- Analytical chemistry
- Chemical compounds
- Materials and material systems
- Chemical kinetics and dynamics
Latest Physics Jobs
- Online ISSN 1089-7690
- Print ISSN 0021-9606
- For Researchers
- For Librarians
- For Advertisers
- Our Publishing Partners
- Physics Today
- Conference Proceedings
- Special Topics
pubs.aip.org
- Privacy Policy
- Terms of Use
Connect with AIP Publishing
This feature is available to subscribers only.
Sign In or Create an Account

Physical Chemistry Chemical Physics

About Physical Chemistry Chemical Physics
High quality research in physical chemistry, chemical physics and biophysical chemistry. Editorial Board Chair: Anouk Rijs Impact factor: 2.9 Time to first decision (peer reviewed only): 34 days
Find an article
Find issues by year (1999 - present), related journals.
- Journal of the Chemical Society, Faraday Transactions (1990-1998)
- Journal of the Chemical Society, Faraday Transactions 1: Physical Chemistry in Condensed Phases (1972-1989)
- Journal of the Chemical Society, Faraday Transactions 2: Molecular and Chemical Physics (1972-1989)
- Transactions of the Faraday Society (1905-1971)
Journal information
- About this Journal
- People and Contacts
- Editorial Board
- Subscription Information
- Follow | | |
Advertisements
Thank you for visiting nature.com. You are using a browser version with limited support for CSS. To obtain the best experience, we recommend you use a more up to date browser (or turn off compatibility mode in Internet Explorer). In the meantime, to ensure continued support, we are displaying the site without styles and JavaScript.
- View all journals
Chemistry articles from across Nature Portfolio
Chemistry is a branch of science that involves the study of the composition, structure and properties of matter. Often known as the central science, it is a creative discipline chiefly concerned with atomic and molecular structure and its change, for instance through chemical reactions.

Reshaping amino acids
Two catalysts working together under light irradiation selectively dehydrogenate proteinogenic amino acids to yield intermediates that can be diversified into non-canonical amino acids.
- Jingyang Qin
- Christopher J. Teskey

Supramolecular crystals for hydrogen storage
Adsorbents for efficient hydrogen storage require both a high gravimetric and volumetric storage capacity. A catenation strategy guided by hydrogen bonding is now demonstrated for the construction of supramolecular crystals with both high volumetric and large gravimetric surface areas, robustness and ideal pore diameters, which contribute to their good performance for hydrogen storage.
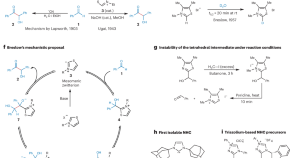
Birth of organocatalysis by N-heterocyclic carbenes
Thiamine, a common enzymatic cofactor, catalyses the benzoin condensation. From 1943, a panoply of mechanistic proposals were invoked to explain the intriguing transformation until two seminal papers by Ronald Breslow about 15 years after the discovery of this reaction helped resolve the mechanistic conundrum and heralded the birth of NHC-organocatalysis.
- Sukriyo Chakraborty
- Akkattu T. Biju
Related Subjects
- Analytical chemistry
- Biochemistry
- Biosynthesis
- Chemical biology
- Chemical education
- Chemical engineering
- Chemical safety
- Cheminformatics
- Chemistry publishing
- Communicating chemistry
- Coordination chemistry
- Electrochemistry
- Environmental chemistry
- Green chemistry
- History of chemistry
- Inorganic chemistry
- Materials chemistry
- Medicinal chemistry
- Nuclear chemistry
- Organic chemistry
- Chemical origin of life
- Photochemistry
- Physical chemistry
- Polymer chemistry
- Process chemistry
- Supramolecular chemistry
- Surface chemistry
- Chemical synthesis
- Theoretical chemistry
Latest Research and Reviews
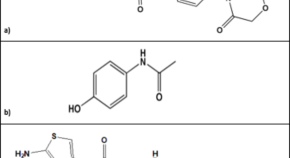
A bioanalytically validated RP-HPLC method for simultaneous quantification of rivaroxaban, paracetamol, and ceftriaxone in human plasma: a combination used for COVID-19 management
- Reham A. Ismail
- Miriam F. Ayad
- Yossra A. Trabik

Imaging in-operando LiCoO 2 nanocrystallites with Bragg coherent X-ray diffraction
Although lithium cobalt(III) oxide (LCO) is a widely used and highly stable lithium-ion battery material, several challenges still need to be overcome. Here, the authors use in-operando Bragg Coherent X-ray Diffraction Imaging to visualize the structural properties of LCO in battery cells and identify inefficiency-increasing domain dynamics during cycling.
- David Serban
- Daniel G. Porter
- Marcus C. Newton
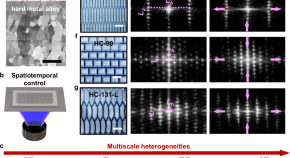
Tailoring smart hydrogels through manipulation of heterogeneous subdomains
Replicating the cellular structures in soft tissue has promise in the design of soft materials. Here, the authors report a hydrogel system with cellular patterns in which strain-driven heterogeneous subdomains are induced, allowing local and global behaviour to be tuned.
- Haoqing Yang
- Tengxiao Liu
- Hongtao Sun
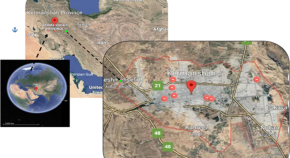
Hospital waste management system in Kermanshah: challenges, future and sustainable management with circular economy
- Mojgan Alighardashi
- Seyyed Alireza Mousavi
- Parviz Mohammadi

Beyond traditional magnetic resonance processing with artificial intelligence
Smart signal processing approaches using Artificial Intelligence are gaining momentum in NMR applications. Here, the authors use AI for quadrature detection using only (Anti-)Echo modulation, accessing uncertainties of signal intensities at each point in a spectrum processed by any given method, and to define a reference-free score of NMR spectrum quality.
- Amir Jahangiri
- Vladislav Orekhov
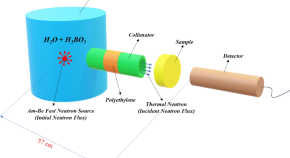
Preparation and characterization of a new Gd 2 O 3 -epoxy composite for neutron shielding applications
- Seyed Mohammadreza Safavi
- Mohammad Outokesh
- Seyed Sajad Jabalamelian
News and Comment
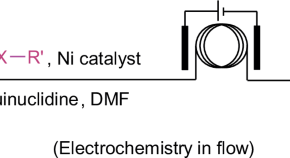
Electrochemical etherification and amination
- Peter W. Seavill

Predicting enantioselectivity for diverse substrates
- Alison Stoddart
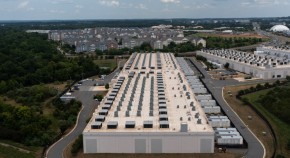
Will AI’s huge energy demands spur a nuclear renaissance?
Contracts with Google and Amazon could help, but bringing new types of reactor online will take larger investments — and time.
- Davide Castelvecchi
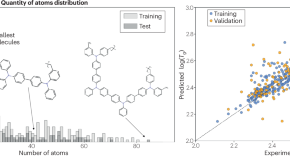
An algorithmic looking glass for transitions
Glass transition temperatures are determined through, for instance, calorimetry, but maybe machine learning models can predict them. Here, researchers test this idea with published data as input for the model, to find a close correlation between predicted and experimental values.
- Alexander Rosu-Finsen
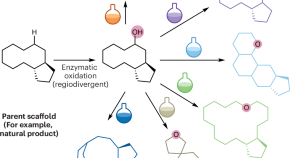
Handles for complexity building
- Francesco Zamberlan
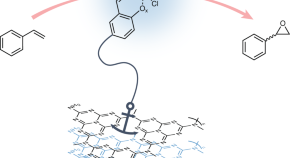
Anchored epoxidation
- Benjamin Martindale
Quick links
- Explore articles by subject
- Guide to authors
- Editorial policies

Share Options
- Share to Facebook
- Share to Linkedin
UVA Researchers Develop New Coatings to Boost Turbine Engine Efficiency
Innovative Materials Could Lead to Cleaner Energy and Lower Costs
A University of Virginia-led research team has developed new protective coatings that allow turbine engines to run at higher temperatures before components begin to fail.
“Hotter engines are more efficient,” said Elizabeth J. Opila , professor and chair of the Department of Materials Science and Engineering at UVA and a lead researcher on the project.
Turbine engines are known for aircraft propulsion, but stationary turbines have many industrial uses, including power generation. They burn fuel to rotate turbine blades, converting mechanical energy to electricity.
“You get more work output per heat input at higher temperatures,” Opila said. “The potential benefits drive interest in coatings that act as a barrier against the reactive gases produced by combustion at these high temperatures that can damage turbine blades.”
Efficiency translates to less fuel consumption and reduced emissions and operating costs — which helps account for why the U.S. Department of Energy’s ARPA-E ULTIMATE program funded the team’s work. They published their findings in the October print issue of Scripta Materialia.
Limits of Today’s High-Temperature Materials
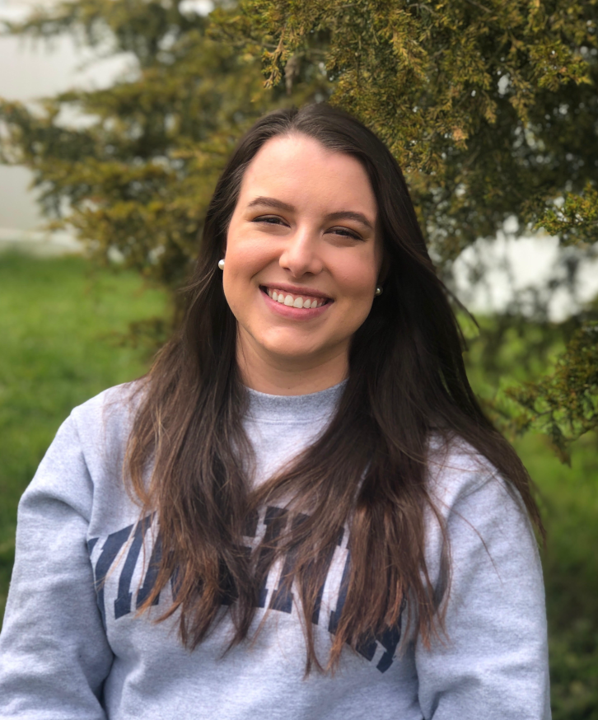
Two primary material systems are used in the hot section of turbine engines today:
- Coated nickel-based superalloys can tolerate up to about 2,200°F — well short of the DOE’s goal of nearly 3,300°F.
- Ceramic composites use several coating layers to protect against degradation from oxidation, a chemical reaction that occurs with exposure to air and moisture. However, these systems are limited by the melting temperature of one layer, silicon, which melts at 2,577°F.
The UVA-led team focused on another material option called refractory metal alloys. Refractory metals were studied extensively in the 1960s. While durable and heat-resistant, they were abandoned due to poor oxidation resistance.
To protect the alloy, the researchers experimented with rare earth oxides — chemical compounds that naturally possess strong protective properties — to come up with one do-it-all coating.
“By combining multiple rare earth oxides, tailoring properties to better protect the underlying substrate can be achieved with just a single layer,” said Kristyn Ardrey, a Ph.D. alumna of Opila’s lab and first author of the paper. “This allowed us to achieve better performance without complex multi-layer coatings.”
A Multidisciplinary Team Approach
Opila’s lab created and tested new combinations of rare earth elements, such as yttrium, erbium and ytterbium. To predict the best combinations and improve performance, they worked with UVA associate professors Bi-Cheng Zhou and Prasanna Balachandran , whose labs specialize in computer simulations and machine learning, a form of artificial intelligence.
The team applied the coatings to alloys using two standard manufacturing methods. One technique heats the material to a molten state before spraying on the surface. The other is applied as a liquid mixture that dries and hardens. The researchers tested and compared how well each method performed under extreme heat and reactive conditions, such as exposure to high-temperature steam.
By combining multiple rare earth oxides, tailoring properties to better protect the underlying substrate can be achieved with just a single layer.
They also partnered with UVA Professor Patrick Hopkin s' ExSiTE Lab, which specializes in using lasers to measure heat resistance and material strength.
“This was a collaborative effort,” Opila said. “Using machine learning and computational methods allowed us to explore a huge range of possible material combinations, and Patrick’s lab was key to understanding the physical characteristics of the materials we developed.”
More Work To Be Done
As one of the first research groups to experiment with multicomponent rare earth oxides, the team knows more testing and refinement are needed. Using computer simulations will help them continue improving the coatings and analyze the best ways to apply them.
But their results represent an important step forward in turbine engine technology — and that’s good for everyone.
“Reducing fuel consumption and emissions while improving engine performance is not only good for industries like energy and aviation,” Opila said. “It also means a cleaner environment and lower costs for everyday consumers.“
Publication
“ Opportunities for novel refractory alloy thermal/environmental barrier coatings using multicomponent rare earth oxides ” was first published online June 4, 2024.
Additional UVA contributors include Mackenzie J. Ridley, Kang Wang, William Riffe, Mukil Ayyasamy and Mahboobe Jassas, representing the departments of Materials Science and Engineering, Mechanical and Aerospace Engineering, and Physics. Kevin Reuwer, Giavanna Angelo and Carolina Tallon of the Department of Materials Science and Engineering at Virginia Tech and Kevin Childrey and Jonathan Laurer of the Commonwealth Center for Advanced Manufacturing also were collaborators.
The Office of Naval Research provided additional funding.
Diverse Expertise
Want to know more about the researchers in this story and how UVA Engineering is advancing high-temperature materials technology through multi-disciplinary approaches? Follow the links below to visit our faculty lab websites.
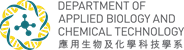
Faculty of Science
Quick Access
- About PolyU
- PolyU A to Z
- Research at PolyU
- Study at PolyU

- Academic Staff
- Adjunct, Honorary and Visiting Staff
- Affiliate Faculty Members
- Research Staff
- Scientific Officers
- Technical Staff
- Administrative Staff
- Bachelor of Science (Honours) Scheme in Biotechnology and Chemical Technology (2025/26 intake onwards)
- Bachelor of Science (Honours) Scheme in Biotechnology and Chemical Technology (2024/25 intake)
- Bachelor of Science (Honours) Scheme in Biotechnology, Food Safety and Chemical Technology Before 2023-24 intake
- Bachelor of Science (Honours) in Applied Biology with Biotechnology
- Bachelor of Science (Honours) in Analytical Sciences for Testing and Certification
- Bachelor of Science (Honours) in Chemical Technology
- List of all Subjects
- Academic Advising
- Academic Exchange and Overseas Internship
- Master of Science in Biopharmaceutical Development and Commercialization
- Master of Science in Sustainable Technology for Carbon Neutrality
- Research Postgraduate Programme
- Postgraduate Exchange
- Postgraduate Studentship and Scholarship
- Record of Training
- Internship Opportunities
- Research Overview
- Knowledge Transfer
- Lo Ka Chung Research Centre for Natural Anti-Cancer Drug Development
State Key Laboratory of Chemical Biology and Drug Discovery (The Hong Kong Polytechnic University)
- Henry Cheng Research Laboratory for Drug Development
- Research Centre for Carbon-Strategic Catalysis
- Teaching Laboratories
- Chromatography
- Mass Spectrometry
- Nuclear Magnetic Resonance Spectroscopy
- Spectroscopy
- X-Ray Crystallography
- Health and Safety
News and Events
- News and Awards
- Photo Gallery
- Video Gallery
- Message from Department
ABCT Portal
- ABCT Staff intranet
- Equipment Master List
- Workflow for Application of Lab Access
- Research Centres
- Academic Advisory Committee
The Department of Applied Biology and Chemical Technology at the Hong Kong Polytechnic University (PolyU) has a long history of interdisciplinary research in chemistry and biology. From 2001 to 2009, with the strong support of the Hong Kong Research Grants Council, the Institute of Molecular Technology for Drug Discovery and Synthesis was established. At the end of 2010, the Partner State Key Laboratory of Chirosciences was approved by the Ministry of Science and Technology (MOST) to be established in PolyU as a partner laboratory of the State Key Laboratory of Bio-organic and Natural Products Chemistry in the Shanghai Institute of Organic Chemistry of the Chinese Academy of Sciences. In mid-2011, the Partner State Key Laboratory of Chirosciences started to operate. In 2018, upon approval by MOST, the Partner State Key Laboratory of Chirosciences was renamed as the State Key Laboratory of Chemical Biology and Drug Discovery.
Since its inception in 2010, the State Key Laboratory of Chemical Biology and Drug Discovery (formerly as Partner State Key Laboratory of Chirosciences) has been focused on researches of organic synthesis, catalysis, chemical biology and drug discovery. There are three major objectives:
- To synthesize and develop novel compounds and natural products as drug candidates;
- To conduct research and develop new pharmaceuticals and health products;
- To explore and develop new molecular techniques for drug discovery.
Research conducted in our laboratory includes:
- Organic synthesis and catalysis on novel molecules and natural products for drug development;
- Discovery and screening of drug candidates, including synthetic compounds and natural products;
- Study of the interaction between small molecules and biomolecules;
- Development of research methods to understand the role of small molecules and biomolecules in biological processes.
The laboratory strives for the following goals:
- To conduct world-class research in chemical biology and drug discovery;
- To nurture outstanding young scientists;
- To enhance knowledge and technology transfer for the chemical and pharmaceutical industry.
香港理工大学应用生物及化学科技学系在化学和生物学跨学科的领域上有着悠久的历史。2001年到2009年期间, 在香港研究资助局大力支持下,分子科技研究所成立了。2010年年底,理工大学获国家科学技术部批准成立手性科学国家重点实验室伙伴实验室,与中科院上海有机所生命有机化学国家重点实验室成为伙伴实验室, 並在2011 年中正式开始运作。及后于2018年获国家科学技术部批准正名为化学生物学及药物研发国家重点实验室。
香港理工大学化学生物学及药物研发国家重点实验室(前称手性科学国家重点实验室伙伴实验室)自2010年成立以来,一直专注于有机合成和催化﹑化学生物学以及相关的诊断学等方面的研究课题。我们实验室订立的研究目标主要有以下三项:
- 研究合成新型化合物和天然产物以及生物化合物的新技术;
- 研发用于提高医疗﹑降低毒性的保健和新型药物制剂;
- 探索和开发新型分子技术用于药物和医疗保健产品的研发。
我们实验室在未来的发展领域将包括以下几个方面:
- 有机合成及催化,尤其是一些新型分子和天然产物的合成以及催化合成;
- 发现和筛选候选药物,包括合成的化合物﹑天然产物及中药里的有效成分;
- 研究小分子和生物体系的相互作用;
- 发展小分子和生物分子作用的研究方法,研究生物过程。
化学生物学及药物研发国家重点实验室在未来几年将努力达成以下预期的目标:
- 进行世界级的化学生物学和药物研发研究;
- 培养该领域年轻的优秀研究人才;
- 通过提升﹑宣传和技术转让,在制药工业领域产生经济效益。
State Key Laboratory of Chemical Biology and Drug Discovery
The Hong Kong Polytechnic University, Hung Hom, Kowloon, Hong Kong
- 3400 8686 (Tel); 2362 2578 (Fax)
- [email protected]
化学生物学及药物研发国家重点实验室
- 3400 8686 (电话); 2362 2578 (传真)
We use Cookies to give you a better experience on our website. By continuing to browse the site without changing your privacy settings, you are consenting to our use of Cookies. For more information, please see our Privacy Policy Statement .
Your browser is not the latest version. If you continue to browse our website, Some pages may not function properly. You are recommended to upgrade to a newer version or switch to a different browser. A list of the web browsers that we support can be found here
What are you looking for?


- Call For Papers 2023
- Online Submission
- Authors GuideLines
Publication Charge
Registration.
- Certificate Request
Thesis Publication
- Journal Indexing
- How to publish research paper
- Research Paper Topics
Editorial Board
- Peer Review & Publication Policy
- Ethics Policy
- Editorial Scope
- 2024- List of Thesis
- Online Thesis Submission
- Thesis Publication Detail
- January 2024 Edition
- February 2024 Edition
- March 2024 Edition
- April 2024 Edition
- May 2024 Edition
- June 2024 Edition
- July 2024 Edition
- August 2024 Edition
- September 2024 Edition
- October 2024 Edition
- January 2023 Edition
- February 2023 Edition
- March 2023 Edition
- April 2023 Edition
- May 2023 Edition
- June 2023 Edition
- July 2023 Edition
- August 2023 Edition
- September 2023 Edition
- October 2023 Edition
- November 2023 Edition
- December 2023 Edition
- January 2022 Edition
- February 2022 Edition
- March 2022 Edition
- April 2022 Edition
- May 2022 Edition
- June 2022 Edition
- July 2022 Edition
- August 2022 Edition
- September 2022 Edition
- October 2022 Edition
- November 2022 Edition
- December 2022 Edition
- January 2021 Edition
- February 2021 Edition
- March 2021 Edition
- April 2021 Edition
- May 2021 Edition
- June 2021 Edition
- July 2021 Edition
- August 2021 Edition
- September 2021 Edition
- October 2021 Edition
- November 2021 Edition
- December 2021 Edition
- January 2020 Edition
- February 2020 Edition
- March 2020 Edition
- April 2020 Edition
- May 2020 Edition
- June 2020 Edition
- July 2020 Edition
- August 2020 Edition
- September 2020 Edition
- October 2020 Edition
- November 2020 Edition
- December 2020 Edition
- January 2019 Edition
- February 2019 Edition
- March 2019 Edition
- April 2019 Edition
- May 2019 Edition
- June 2019 Edition
- July 2019 Edition
- August 2019 Edition
- September 2019 Edition
- October 2019 Edition
- November 2019 Edition
- December 2019 Edition
- January 2018 Edition
- February 2018 Edition
- March 2018 Edition
- April 2018 Edition
- May 2018 Edition
- June 2018 Edition
- July 2018 Edition
- August 2018 Edition
- September 2018 Edition
- October 2018 Edition
- November 2018 Edition
- December 2018 Edition
- January 2017 Edition
- February 2017 Edition
- March 2017 Edition
- April 2017 Edition
- May 2017 Edition
- June 2017 Edition
- July 2017 Edition
- August 2017 Edition
- September 2017 Edition
- October 2017 Edition
- November 2017 Edition
- December 2017 Edition
- Archives- All Editions
- Registration Form
- Copyright Transfer
- Scopus Indexing
Impact Factor Journal High Citations & Indexed Worldwide
IJSER is an highly indexed research journal, provding DOI for research papers, using DOI your published paper is indexed & accessible from various universities, libraries/repositories.
Part of eco-friendly community journals
Being a part of an eco-friendly community, IJSER favors and promotes e-publication of papers to truly present itself as an online ‘GREEN journal’. Find out more and apply now ...
Aim high with IJSER scholarship & university affiliation program
Journal Associated with leading universities Provide Scholarship to eligible candidates. for Higher education & Research
Submit Your Paper
Research papers, survey papers, case studies, scholarly articles
Details for the thesis publication.
Call of Papers 2023
Paper Submission Deadline : February 28,2023 - Vol 14,Issue 2, February 2023
Highly Qualified and experienced review board
Certificates
Digitally Signed E-certificate & Academic Award Certificate.

January: Vol 14, Issue 1
December: Vol 13, Issue 12
November: Vol 13, Issue 11
October: Vol 13, Issue 10
September: Vol 13, Issue 9
August: Vol 13, Issue 8
“ We are an American based journal with headquarters in the US.”
International journal of scientific & engineering research -ijser (issn 2229-5518) - call for research papers.
The International Journal of Scientific & Engineering Research is a one-stop, open access source for a large number of high quality and peer reviewed journals in all the fields of science, engineering and technology. Scientists and engineers involved in research can make the most of this growing global forum to publish papers covering their original research or extended versions of already published conference/journal papers, scholarly journals, academic articles, etc. The published papers are made highly visible to the scientific community through a wide indexing policy adopted by this online international journal. Hence, they can freely be accessed and utilized by everyone for the development of science and technology. Being a part of an eco-friendly community, IJSER favors and promotes e-publication of papers to truly present itself as an online ‘GREEN journal.’ Submit your papers today!. Read more ...
Research Paper Registration Process for accepted papers
Conferences
List of conferences co-sponsored by IJSER.
We invite you to submit high quality papers for review and possible publication in all areas of engineering, science and technology. All authors must agree on the content of the manuscript and its submission for publication in this journal before it is submitted to us. All the manuscripts submitted for publication are first peer reviewed to make sure they are original, relevant and readable. Manuscripts should be submitted via Online Submission only.
IJSER publishes articles that emphasizes research, development and application within the fields of engineering, science and technology. All manuscripts are pre-reviewed by the editorial review committee. Contributions must be original, not previously or simultaneously published elsewhere, and are critically reviewed before they are published. Papers, which must be written in English, should have sound grammar and proper terminologies.
IJSER is an international online journal in English published monthly.This academic journal and scholarly peer reviewed journal is an online journal having full access to the research and review paper. IJSER hopes that Researchers, Research scholars, Academician, Industrialists, Consultancy etc. would make use of this journal publication for the development of science and technology.
- Conferences 2023

IJSER co-sponsor various conferences worldwide.Conference Sponsorship Program (CSP) is one of the excellent services offered by IJSER that is uniquely intended to support the researchers and conference organizers. CSP provides conference organizers a privileged platform for the publishing of research work presented in conference proceedings. CSP is deliberated to disseminate scientific research and to establish long term International collaborations and partnerships with academic communities and conference organizers.
- Scientists and engineers who want to publish their high quality, original research work online can find International Journal of Scientific and Engineering Research (IJSER) serving their purpose. We cover a broad range of research paper topics listed alphabetically and are quite keen to expand the list further. If you’ve original research papers or extended versions of already published papers, then submit them to us for peer reviewing by the editorial review committee. Once the paper is accepted, it will be published on our website. So, go through the research paper topics and start submitting yours!
Associated with Leading Universities & Institutes
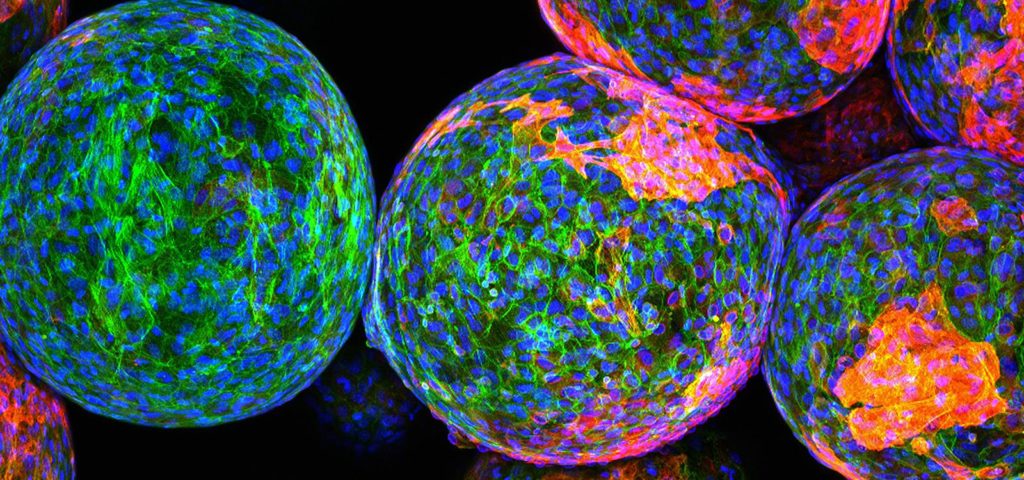
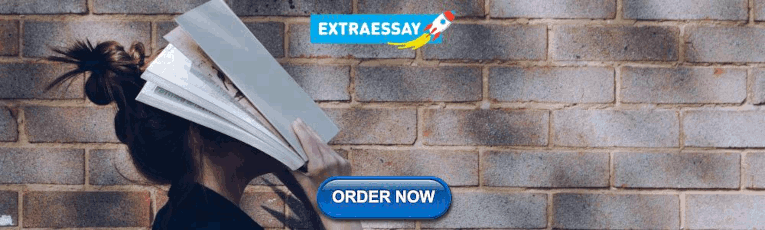
Biomedical and Biotechnology
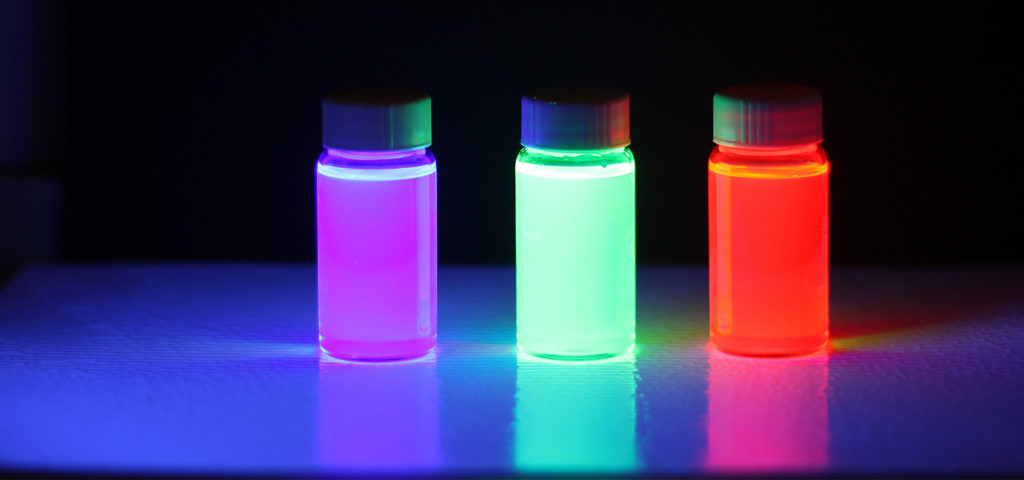
Catalysis and Reaction Engineering
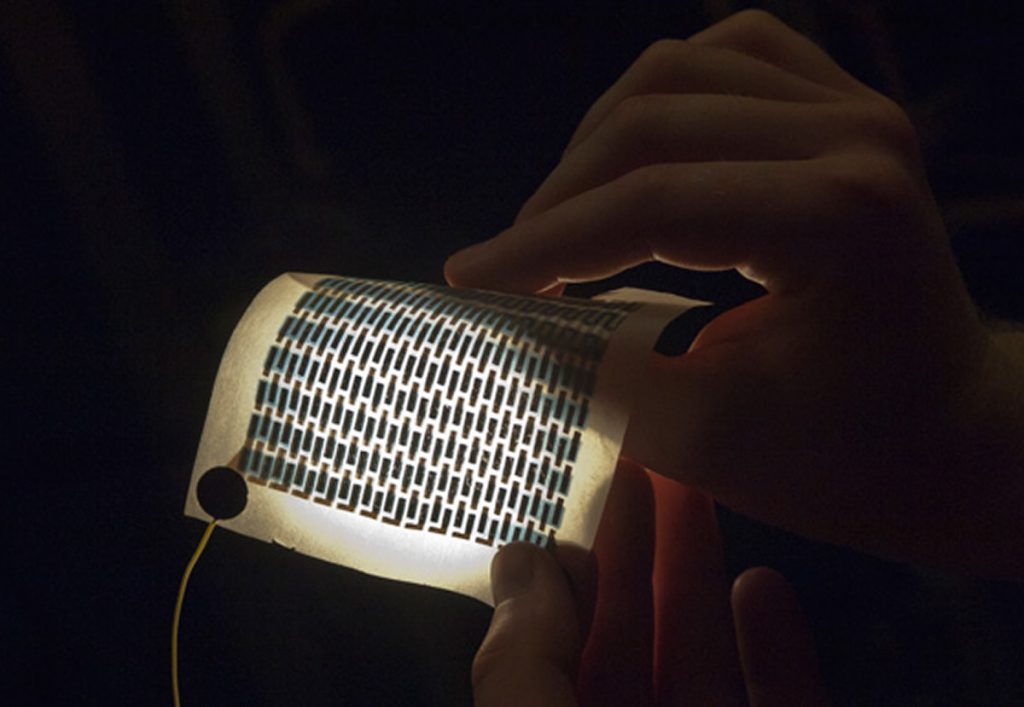
Environment and Sustainability
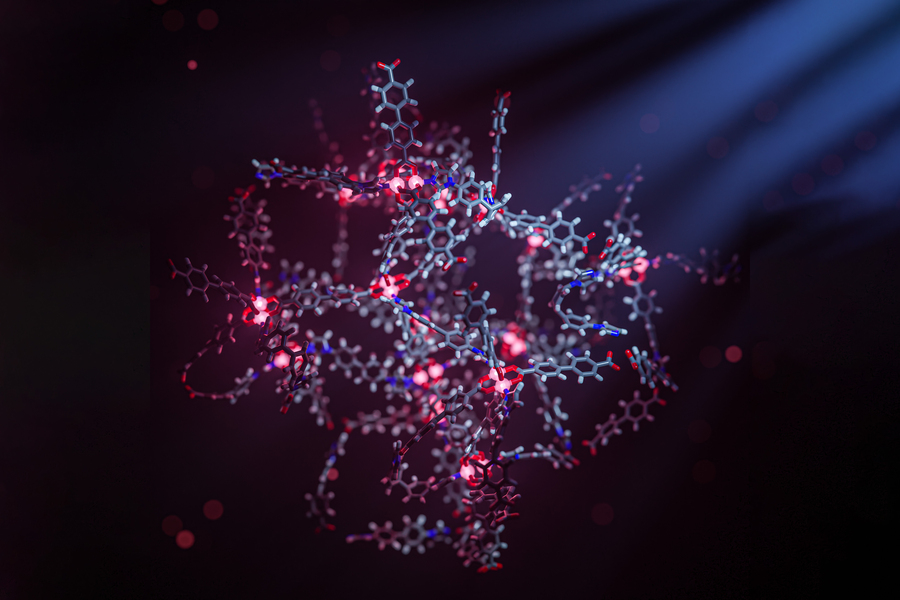
Math and Computational Systems
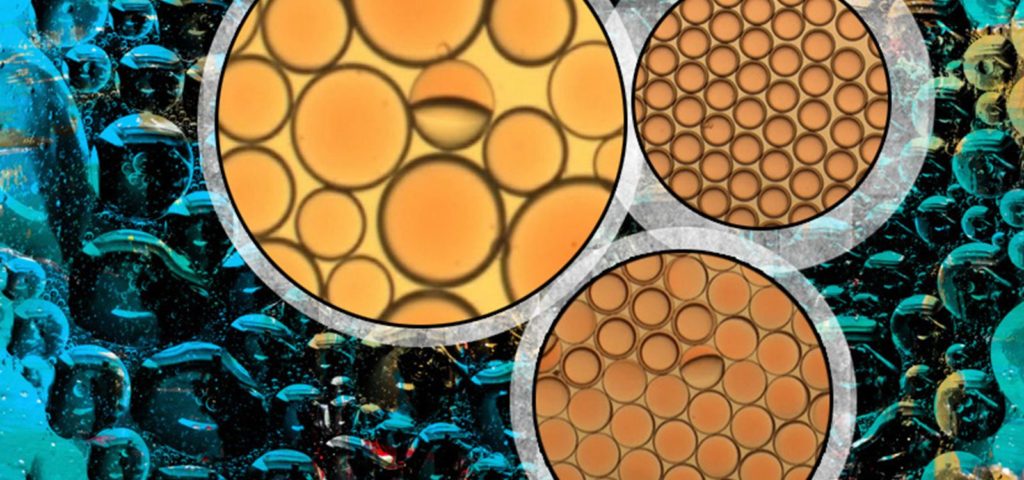
Transport and Thermodynamics
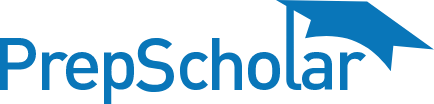
Choose Your Test
- Search Blogs By Category
- College Admissions
- AP and IB Exams
- GPA and Coursework
113 Great Research Paper Topics
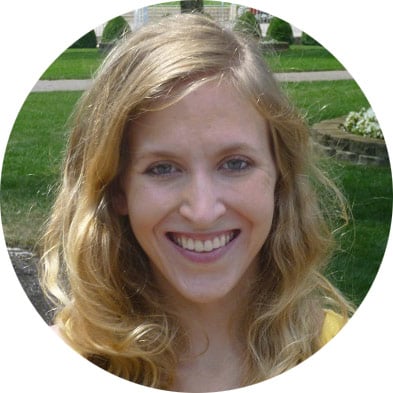
General Education
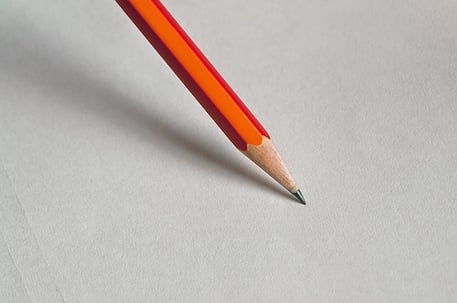
One of the hardest parts of writing a research paper can be just finding a good topic to write about. Fortunately we've done the hard work for you and have compiled a list of 113 interesting research paper topics. They've been organized into ten categories and cover a wide range of subjects so you can easily find the best topic for you.
In addition to the list of good research topics, we've included advice on what makes a good research paper topic and how you can use your topic to start writing a great paper.
What Makes a Good Research Paper Topic?
Not all research paper topics are created equal, and you want to make sure you choose a great topic before you start writing. Below are the three most important factors to consider to make sure you choose the best research paper topics.
#1: It's Something You're Interested In
A paper is always easier to write if you're interested in the topic, and you'll be more motivated to do in-depth research and write a paper that really covers the entire subject. Even if a certain research paper topic is getting a lot of buzz right now or other people seem interested in writing about it, don't feel tempted to make it your topic unless you genuinely have some sort of interest in it as well.
#2: There's Enough Information to Write a Paper
Even if you come up with the absolute best research paper topic and you're so excited to write about it, you won't be able to produce a good paper if there isn't enough research about the topic. This can happen for very specific or specialized topics, as well as topics that are too new to have enough research done on them at the moment. Easy research paper topics will always be topics with enough information to write a full-length paper.
Trying to write a research paper on a topic that doesn't have much research on it is incredibly hard, so before you decide on a topic, do a bit of preliminary searching and make sure you'll have all the information you need to write your paper.
#3: It Fits Your Teacher's Guidelines
Don't get so carried away looking at lists of research paper topics that you forget any requirements or restrictions your teacher may have put on research topic ideas. If you're writing a research paper on a health-related topic, deciding to write about the impact of rap on the music scene probably won't be allowed, but there may be some sort of leeway. For example, if you're really interested in current events but your teacher wants you to write a research paper on a history topic, you may be able to choose a topic that fits both categories, like exploring the relationship between the US and North Korea. No matter what, always get your research paper topic approved by your teacher first before you begin writing.
113 Good Research Paper Topics
Below are 113 good research topics to help you get you started on your paper. We've organized them into ten categories to make it easier to find the type of research paper topics you're looking for.
Arts/Culture
- Discuss the main differences in art from the Italian Renaissance and the Northern Renaissance .
- Analyze the impact a famous artist had on the world.
- How is sexism portrayed in different types of media (music, film, video games, etc.)? Has the amount/type of sexism changed over the years?
- How has the music of slaves brought over from Africa shaped modern American music?
- How has rap music evolved in the past decade?
- How has the portrayal of minorities in the media changed?
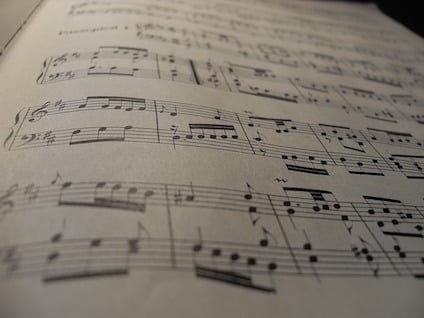
Current Events
- What have been the impacts of China's one child policy?
- How have the goals of feminists changed over the decades?
- How has the Trump presidency changed international relations?
- Analyze the history of the relationship between the United States and North Korea.
- What factors contributed to the current decline in the rate of unemployment?
- What have been the impacts of states which have increased their minimum wage?
- How do US immigration laws compare to immigration laws of other countries?
- How have the US's immigration laws changed in the past few years/decades?
- How has the Black Lives Matter movement affected discussions and view about racism in the US?
- What impact has the Affordable Care Act had on healthcare in the US?
- What factors contributed to the UK deciding to leave the EU (Brexit)?
- What factors contributed to China becoming an economic power?
- Discuss the history of Bitcoin or other cryptocurrencies (some of which tokenize the S&P 500 Index on the blockchain) .
- Do students in schools that eliminate grades do better in college and their careers?
- Do students from wealthier backgrounds score higher on standardized tests?
- Do students who receive free meals at school get higher grades compared to when they weren't receiving a free meal?
- Do students who attend charter schools score higher on standardized tests than students in public schools?
- Do students learn better in same-sex classrooms?
- How does giving each student access to an iPad or laptop affect their studies?
- What are the benefits and drawbacks of the Montessori Method ?
- Do children who attend preschool do better in school later on?
- What was the impact of the No Child Left Behind act?
- How does the US education system compare to education systems in other countries?
- What impact does mandatory physical education classes have on students' health?
- Which methods are most effective at reducing bullying in schools?
- Do homeschoolers who attend college do as well as students who attended traditional schools?
- Does offering tenure increase or decrease quality of teaching?
- How does college debt affect future life choices of students?
- Should graduate students be able to form unions?
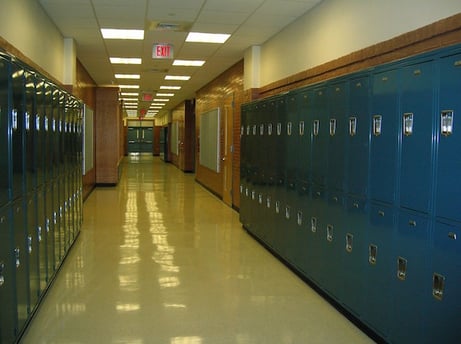
- What are different ways to lower gun-related deaths in the US?
- How and why have divorce rates changed over time?
- Is affirmative action still necessary in education and/or the workplace?
- Should physician-assisted suicide be legal?
- How has stem cell research impacted the medical field?
- How can human trafficking be reduced in the United States/world?
- Should people be able to donate organs in exchange for money?
- Which types of juvenile punishment have proven most effective at preventing future crimes?
- Has the increase in US airport security made passengers safer?
- Analyze the immigration policies of certain countries and how they are similar and different from one another.
- Several states have legalized recreational marijuana. What positive and negative impacts have they experienced as a result?
- Do tariffs increase the number of domestic jobs?
- Which prison reforms have proven most effective?
- Should governments be able to censor certain information on the internet?
- Which methods/programs have been most effective at reducing teen pregnancy?
- What are the benefits and drawbacks of the Keto diet?
- How effective are different exercise regimes for losing weight and maintaining weight loss?
- How do the healthcare plans of various countries differ from each other?
- What are the most effective ways to treat depression ?
- What are the pros and cons of genetically modified foods?
- Which methods are most effective for improving memory?
- What can be done to lower healthcare costs in the US?
- What factors contributed to the current opioid crisis?
- Analyze the history and impact of the HIV/AIDS epidemic .
- Are low-carbohydrate or low-fat diets more effective for weight loss?
- How much exercise should the average adult be getting each week?
- Which methods are most effective to get parents to vaccinate their children?
- What are the pros and cons of clean needle programs?
- How does stress affect the body?
- Discuss the history of the conflict between Israel and the Palestinians.
- What were the causes and effects of the Salem Witch Trials?
- Who was responsible for the Iran-Contra situation?
- How has New Orleans and the government's response to natural disasters changed since Hurricane Katrina?
- What events led to the fall of the Roman Empire?
- What were the impacts of British rule in India ?
- Was the atomic bombing of Hiroshima and Nagasaki necessary?
- What were the successes and failures of the women's suffrage movement in the United States?
- What were the causes of the Civil War?
- How did Abraham Lincoln's assassination impact the country and reconstruction after the Civil War?
- Which factors contributed to the colonies winning the American Revolution?
- What caused Hitler's rise to power?
- Discuss how a specific invention impacted history.
- What led to Cleopatra's fall as ruler of Egypt?
- How has Japan changed and evolved over the centuries?
- What were the causes of the Rwandan genocide ?
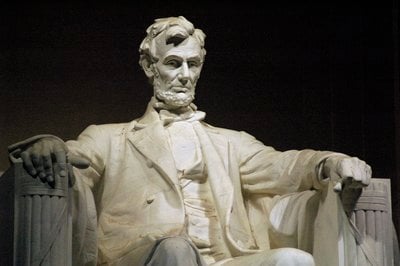
- Why did Martin Luther decide to split with the Catholic Church?
- Analyze the history and impact of a well-known cult (Jonestown, Manson family, etc.)
- How did the sexual abuse scandal impact how people view the Catholic Church?
- How has the Catholic church's power changed over the past decades/centuries?
- What are the causes behind the rise in atheism/ agnosticism in the United States?
- What were the influences in Siddhartha's life resulted in him becoming the Buddha?
- How has media portrayal of Islam/Muslims changed since September 11th?
Science/Environment
- How has the earth's climate changed in the past few decades?
- How has the use and elimination of DDT affected bird populations in the US?
- Analyze how the number and severity of natural disasters have increased in the past few decades.
- Analyze deforestation rates in a certain area or globally over a period of time.
- How have past oil spills changed regulations and cleanup methods?
- How has the Flint water crisis changed water regulation safety?
- What are the pros and cons of fracking?
- What impact has the Paris Climate Agreement had so far?
- What have NASA's biggest successes and failures been?
- How can we improve access to clean water around the world?
- Does ecotourism actually have a positive impact on the environment?
- Should the US rely on nuclear energy more?
- What can be done to save amphibian species currently at risk of extinction?
- What impact has climate change had on coral reefs?
- How are black holes created?
- Are teens who spend more time on social media more likely to suffer anxiety and/or depression?
- How will the loss of net neutrality affect internet users?
- Analyze the history and progress of self-driving vehicles.
- How has the use of drones changed surveillance and warfare methods?
- Has social media made people more or less connected?
- What progress has currently been made with artificial intelligence ?
- Do smartphones increase or decrease workplace productivity?
- What are the most effective ways to use technology in the classroom?
- How is Google search affecting our intelligence?
- When is the best age for a child to begin owning a smartphone?
- Has frequent texting reduced teen literacy rates?
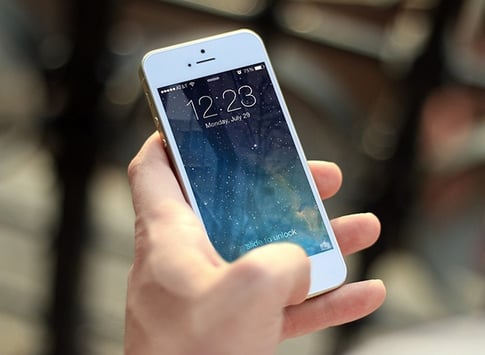
How to Write a Great Research Paper
Even great research paper topics won't give you a great research paper if you don't hone your topic before and during the writing process. Follow these three tips to turn good research paper topics into great papers.
#1: Figure Out Your Thesis Early
Before you start writing a single word of your paper, you first need to know what your thesis will be. Your thesis is a statement that explains what you intend to prove/show in your paper. Every sentence in your research paper will relate back to your thesis, so you don't want to start writing without it!
As some examples, if you're writing a research paper on if students learn better in same-sex classrooms, your thesis might be "Research has shown that elementary-age students in same-sex classrooms score higher on standardized tests and report feeling more comfortable in the classroom."
If you're writing a paper on the causes of the Civil War, your thesis might be "While the dispute between the North and South over slavery is the most well-known cause of the Civil War, other key causes include differences in the economies of the North and South, states' rights, and territorial expansion."
#2: Back Every Statement Up With Research
Remember, this is a research paper you're writing, so you'll need to use lots of research to make your points. Every statement you give must be backed up with research, properly cited the way your teacher requested. You're allowed to include opinions of your own, but they must also be supported by the research you give.
#3: Do Your Research Before You Begin Writing
You don't want to start writing your research paper and then learn that there isn't enough research to back up the points you're making, or, even worse, that the research contradicts the points you're trying to make!
Get most of your research on your good research topics done before you begin writing. Then use the research you've collected to create a rough outline of what your paper will cover and the key points you're going to make. This will help keep your paper clear and organized, and it'll ensure you have enough research to produce a strong paper.
What's Next?
Are you also learning about dynamic equilibrium in your science class? We break this sometimes tricky concept down so it's easy to understand in our complete guide to dynamic equilibrium .
Thinking about becoming a nurse practitioner? Nurse practitioners have one of the fastest growing careers in the country, and we have all the information you need to know about what to expect from nurse practitioner school .
Want to know the fastest and easiest ways to convert between Fahrenheit and Celsius? We've got you covered! Check out our guide to the best ways to convert Celsius to Fahrenheit (or vice versa).
These recommendations are based solely on our knowledge and experience. If you purchase an item through one of our links, PrepScholar may receive a commission.
Trending Now
How to Get Into Harvard and the Ivy League
How to Get a Perfect 4.0 GPA
How to Write an Amazing College Essay
What Exactly Are Colleges Looking For?
ACT vs. SAT: Which Test Should You Take?
When should you take the SAT or ACT?
Get Your Free
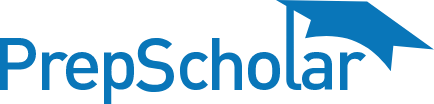
Find Your Target SAT Score
Free Complete Official SAT Practice Tests
How to Get a Perfect SAT Score, by an Expert Full Scorer
Score 800 on SAT Math
Score 800 on SAT Reading and Writing
How to Improve Your Low SAT Score
Score 600 on SAT Math
Score 600 on SAT Reading and Writing
Find Your Target ACT Score
Complete Official Free ACT Practice Tests
How to Get a Perfect ACT Score, by a 36 Full Scorer
Get a 36 on ACT English
Get a 36 on ACT Math
Get a 36 on ACT Reading
Get a 36 on ACT Science
How to Improve Your Low ACT Score
Get a 24 on ACT English
Get a 24 on ACT Math
Get a 24 on ACT Reading
Get a 24 on ACT Science
Stay Informed
Get the latest articles and test prep tips!
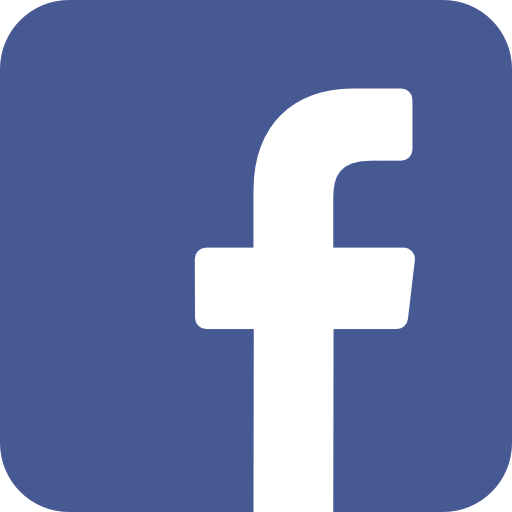
Christine graduated from Michigan State University with degrees in Environmental Biology and Geography and received her Master's from Duke University. In high school she scored in the 99th percentile on the SAT and was named a National Merit Finalist. She has taught English and biology in several countries.
Ask a Question Below
Have any questions about this article or other topics? Ask below and we'll reply!
Chemical Technology Research Paper Topics

This collection of chemical technology research paper topics provides the list of 25 potential topics for research papers and an overview article about history of chemical industry and technology.
1. Biopolymers
Biopolymers are natural polymers, long-chained molecules (macromolecules) consisting mostly of a repeated composition of building blocks or monomers that are formed and utilized by living organisms. Each group of biopolymers is composed of different building blocks, for example chains of sugar molecules form starch (a polysaccharide), chains of amino acids form proteins and peptides, and chains of nucleic acid form DNA and RNA (polynucleotides). Biopolymers can form gels, fibers, coatings, and films depending on the specific polymer, and serve a variety of critical functions for cells and organisms. Advances in metabolic engineering, environmental considerations about renewable polymers from nonpetroleum feedstocks, and the expansion in molecular biology and protein engineering tools in general are taking biopolymer synthesis and production in new directions. The opportunity to enhance, alter, or direct the structural features of biopolymers through genetic manipulation, physiological controls, or enzymatic processes provides new routes to novel polymers with specialty functions. The use of biopolymers in commodity and specialty materials, as well as biomedical applications, can be expected to continue to increase with respect to petrochemical-derived materials. The benefit in tailoring structural features is a plus for generating higher performance properties or more specialized functional performance. Biosynthesis and disposal of biopolymers can be considered within a renewable resource loop, reducing environmental burdens associated with synthetic polymers derived from petrochemicals that often require hundreds of years to degrade. In addition, biopolymers can often be produced from low cost agricultural feedstocks versus petroleum supplies and thereby generate value-added products.
Academic Writing, Editing, Proofreading, And Problem Solving Services
Get 10% off with 24start discount code.
Boranes are chemical compounds of boron and hydrogen. During the 1950s, the U.S. government sponsored a major secretive effort to produce rocket and aircraft fuels based on boron hydrides. Much of the information initially available to the U.S. effort was contained in a book written by the German chemist Alfred Stock in 1933. When burned in air, the energy released by various boron hydride compounds, as measured by their heat of combustion, is 20 to 55 percent greater than the energy released by petroleum-based jet fuels. It was expected that this greater energy content of the boron fuels would translate into equivalent higher payloads or ranges for rockets and aircraft. All of the boron fuel manufacturing processes started with the production of diborane, a compound composed of two boron atoms linked to six hydrogen atoms. Initially, this was produced by reacting lithium hydride with boron trifluoride or boron trichloride in diethyl ether as a solvent. This entailed a need to recover and recycle the expensive lithium. A later process produced diborane by reacting sodium borohydride with boron trichloride in the presence of catalytic amounts of aluminum chloride, using a solvent called diglyme.
3. Chemical Process Engineering
The chemical industry expanded dramatically during the twentieth century to become a highly integrated and increasingly influential contributor to the international economy. Its products seeded and fertilized the growth of other new technologies, particularly in the textiles, explosives, transport, and pharmaceutical industries. The industry also became a major supporter of industrial research, especially in the U.S. and Germany. The production of chemicals during the century can be described as a history of products, processes and professions.
The larger scale changes in chemical production can be better understood in terms of processes rather than as discrete products. Indeed, Hardie and Pratt (1966) describe the history of the chemical industry in terms of the history of its processes; that is, the succession of actions that transform raw materials into a new chemical product. Such conversion may involve chemical reactions (e.g., the production of soda alkali and sulfuric acid in the LeBlanc process); physical change (e.g., oxidation by roasting, or distillation by boiling and condensation); or physical manipulation (e.g., by grinding, mixing and extruding).
4. Chemical Warfare
Popular fiction forecast the use of poison gas in warfare from the 1890s. While an effort was made to ban the wartime use of gas at The Hague International Peace Conference in 1899, military strategists and tacticians dismissed chemical weapons as a fanciful notion. The stalemate of World War I changed this mindset. Under Fritz Haber, a chemist at the Kaiser Wilhelm Institute, Germany’s chemical industry began making gas weapons. Compressed chlorine gas in 5730 cylinders was released against French Algerian and Canadian troops at Ypres, Belgium, on April 22, 1915. The gas attack resulted in approximately 3000 casualties, including some 800 deaths. Within months the British and French developed both gas agents of their own and protective gear, ensuring that chemical warfare would become a regular feature of the war.
A variety of lethal and nonlethal chemical agents were developed in World War I. Lethal agents included the asphyxiating gases such as chlorine, phosgene, and diphosgene that drown their victims in mucous, choking off the supply of oxygen from the lungs. A second type were blood gases like hydrogen cyanide, which block the body’s ability to absorb oxygen from red corpuscles. Incapacitating gases included lachrymatorics (tear gases) and vesicants (blistering gases). The most notorious of these is mustard gas (Bis-[2- chloroethyl] sulphide), a blistering agent that produces horrible burns on the exposed skin and destroys mucous tissue and also persists on the soil for as long as 48 hours after its initial dispersion.
5. Chromatography
The natural world is one of complex mixtures, often with up to a 100,000 (e.g., proteins in the human body) or 1,000,000 (e.g., petroleum) components. Separation methods necessary to cope with these, and with the simpler but still challenging mixtures encountered, for example in pharmaceutical analysis, are based on chromatography and electrophoresis, and underpin research, development, and quality control in numerous industries and in environmental, food, and forensic analysis. In chromatography, a sample is dissolved in a mobile phase (initially this was a liquid), which is then passed through a stationary phase (which is either a liquid or a solid) held in a small diameter tube—the ‘‘column.’’ According to the differing relative solubilities in the two phases, mixture components travel through the column at different rates and become separated before emerging and detected by the measurement of some chemical or physical property. The sample size can be as small as one picogram (10–12 g), but tens of grams can be handled in preparative separations.
6. Coatings, Pigments, and Paints
The development and application of pigments, paints, and coatings have been an integral part of human development from Paleolithic cave paintings, the art of early civilizations, and protection of buildings from rain. During the twentieth century, understanding of chemicals and the manufacturing need for high-quality decorative and protective coatings drove rapid progression in paint technology. All paints employ the same basic ingredients: pigment to provide color; a medium to bind or suspend the pigment, including emulsions such as resins or oils; and a solvent carrier, which acts to wet the surface to ensure adhesion and thins the resin to make it easy to apply. Early pigments such as those used in Minoan frescoes and Anasazi rock art and the use of henna as body paint originated primarily from natural sources. Clays, mineral pigments such as iron or chromium oxide, vegetable dyes, animal sources such as shells or urine, as well as precious metals and gems gave rise to a broad selection of pigments that were often unique to one region (e.g., lapis lazuli) and thus highly prized as trade items. But value wasn’t limited merely to pigments. Preservation of painted surfaces required protective resins. The most successful early coating was lacquer, used in China since at least the 1300 BC. Lacquer was processed—using a highly guarded secret formula—from resin from the lacquer tree (Rhus vernicflua). Shellac, produced from the gum secreted by an insect native to India and southern Asia, makes a varnish when mixed with acetone or alcohol and was used from the eighteenth century. Natural plant resins dissolved in oil or solvent such as turpentine were used in the nineteenth century— evaporation of the solvent leaves a lacquer coating.
7. Combinatorial Chemistry
Combinatorial chemistry is a term created about 1990 to describe the rapid generation of multitudes of chemical structures with the main focus on discovering new drugs. In combinatorial chemistry the chemist should perform at least one step of the synthesis in combinatorial fashion. In the classical chemical synthesis, one synthetic vessel (flask, reactor) is used to perform chemical reaction designed to create one chemical entity. Combinatorial techniques utilize the fact that several operations of the synthesis can be performed simultaneously. Historically, the first papers bringing the world’s attention to combinatorial chemistry were published in 1991, but none of these papers used the term combinatorial chemistry. Interestingly, they were not the first papers describing the techniques for preparation of compound mixtures for biological evaluation. Previously, H. Mario Geysen’s lab had prepared mixtures of peptides for identification of antibody ligands in 1986. Other laboratories heavily engaged in synthesizing multitudes or mixtures of peptides were Richard A. Houghten’s laboratory in San Diego and A ´ rpa´d Furka’s laboratory in Budapest. The recollections of the authors of these historical papers were published in the journal dedicated to combinatorial chemistry, Journal of Combinatorial Chemistry.
8. Cracking
Three major innovations emerged to meet the dramatically higher needs for quantity and better quality motor fuel in the twentieth century: thermal cracking, tetra ethyl lead, and catalytic cracking (including the fluid method). William M. Burton of Standard Oil, Indiana, in 1911–1913 raised gasoline fractions from petroleum distillation from 15 to 40 percent by increasing both temperatures and pressures. Benjamin Stillman Jr. of Yale University had discovered in 1855 that high temperatures could transform or ‘‘crack’’ heavy petroleum fractions into lighter or volatile components, but at atmospheric pressure over half of the raw material was lost to vaporization before the cracking temperature (about 360C) was reached. English scientists James Dewar and Boverton Redwood discovered and patented in 1889 a process using higher pressure to restrain more of the heavier fractions and increase the volatile components. Burton and his team, not aware of this patent at the start of their work, similarly used higher pressure to improve his gasoline yields. Their process by 1913 employed a drum 9 by 2.5 meters diameter over a furnace and a long runback pipe of 300 millimeter diameter that carried vapors to condensing coils and simultaneously allowed heavier fractions to drip back into the drum for more cracking. A tank connected to the condensing coils separated gasoline from the uncondensed hydrocarbon vapors. They used a comparatively low (5.1 atmospheres) pressure because they relied on riveted plates. Burton’s team improved the batch process in the next three years with false bottom plates in the still to improve cleaning time of coke deposits, and a bubble tower to improve fractionation of cracked vapors and increase gasoline yield. Refinery manager Edgar M. Clark took cracking to the next step by using tubes of about 100 millimeters diameter as the primary contact with the furnace, which increased cracking time and allowed pressures of about 6.8 atmospheres, and decreased maintenance time because of reduced coke deposition and fuel costs.
9. Detergents
Detergents are cleaning agents used to remove foreign matter in suspension from soiled surfaces including human skin, textiles, hard surfaces in the home and metals in engineering. Technically called surfactants, detergents form a surface layer between immiscible phases, facilitating wetting by reducing surface tension. Detergent molecules have two parts, one of which is water-soluble (lyophobic) and the other oil or fat-soluble (lyophilic). They are adsorbed onto surfaces where they remove dirt by suspending it in foam. Some also act as biocides, but no single product does these things equally well. Special detergents also have many uses in industry and engineering. In the oil industry for example, surfactants are sometimes used to promote oil flow in porous rocks, or to flush out oil left behind by a water flood. In this case a band of detergent is put down before the water to create a low surface tension and thus allow the oil-bearing rock to be scrubbed clean. The water is often made viscous by adding a polymer to prevent it breaking through the surfactant layer. Detergents are also widely used in industrial flotation processes to separate lighter particles from a mixture with heavier materials.
The decade commencing in 1900 marked the end of half a century of remarkable inventiveness in synthetic dyestuffs that had started with William Perkin’s 1856 discovery of the aniline dye known as mauve. The products, derived mainly from coal tar hydrocarbons, included azo dyes, those containing the atomic grouping – N = N -, and artificial alizarin (1869–1870) and indigo (1897). By 1900, through intensive research and development, control of patents, and aggressive marketing, the industry was dominated by German manufacturers, such as BASF of Ludwigshafen, and Bayer, of Leverkusen. A new range of dyes based on anthraquinone (from which alizarin and congeners were made), and generally known as vat dyes, were the first major innovations in the twentieth century. Anthraquinone was obtained by oxidation of the three-ring aromatic hydrocarbon anthracene. Vat dyes are generally applied in reduced, soluble form; they then reoxidize to the original pigment and are extremely stable.
11. Electrochemistry
Electrochemistry deals with the relationship between chemical change and electricity. Under normal conditions, a chemical reaction is accompanied by the liberation or absorption of heat and not of any other form of energy. However, there are many so-called electrochemical reactions that when allowed to proceed in contact with two electronic conductors joined by conducting wires, will generate electrical energy in this external circuit. Current between the electrodes (usually metallic plates or rods) is carried by electrons, while in the electrolyte, a nonmetallic ionic compound either in the molten condition or in solution in water or other solvents, ions carry the current. Conversely, the energy of an electric current can be used to bring about many chemical reactions that do not occur spontaneously. The process in which electrical energy is directly converted into chemical energy is called electrolysis. The products of an electrolytic process have a tendency to react spontaneously with one another, reproducing the substances that were reactants and were therefore consumed during the electrolysis. If this reverse reaction is allowed to occur under proper conditions, a large proportion of the electrical energy used in the electrolysis can be regenerated. This possibility is used in accumulators or storage cells, sets of which are known as storage batteries.
12. Electrophoresis
Electrophoresis is a separation technique that involves the migration of charged colloidal particles in a liquid under the influence of an applied electric field. The word is derived from electro, referring to the energy of electricity, and phoresis, from the Greek verb phoros, meaning ‘‘to carry across.’’ Electrophoresis has many applications in analytical chemistry, particularly biochemistry. It is one of the staple tools in molecular biology and it is of critical value in many aspects of genetic manipulation, including DNA studies, and in forensic chemistry. Swedish biochemist Arne Tiselius carried out studies on proteins and colloids in the 1920s, and in 1930 introduced electrophoresis as a new technique for separating proteins in solution on the basis of their electrical charge. Tiselius was awarded the 1948 Nobel Prize in chemistry for this work, and the technique became a common tool in the 1940s and 1950s. Biological molecules such as amino acids, peptides, proteins, nucleotides, and nucleic acids, possess ionizable groups. At any given pH (concentration of hydrogen ions), these molecules exist in solution as electrically charged species either as cations (positive, or þ) or anions (negative, or ). Depending on the nature of the net charge, the charged particles will migrate either to the cathode or to the anode. For example, proteins in an electric field separate according to size, shape, and charge with charges contributed by the side chains of the amino acids composing the proteins. The charge of the protein depends on the hydrogen ion content of the surrounding buffer with a high ionic strength resulting in a greater charge.
13. Environmental Monitoring
As a dynamic system, the environment is changing continually, with feedback from both natural (climatic or biogeochemical) and anthropogenic (human activities) sources. Assessing the rate and magnitude of environmental processes is difficult, especially as data collection over time is limited to the last century, or even the last few decades. Since the 1980s, environmental monitoring programs have been developed as a response to concerns that environmental impact or sustainability of policy initiatives could not be evaluated adequately. So-called State of the Environment Reports date from this period. Many are concerned with the state of national, regional, or local environments (land, rivers, or seas); others focus on environments at particular risk, mostly due to human impact and pollution (e.g., environmental contaminants in marine or terrestrial wildlife), or those where environmental quality is significant in the context of human health (e.g., urban air quality; water resources, fisheries). Many monitoring programs include information collected remotely by satellites (see Satellites, Environmental Sensing), but this entry focuses on technologies and policies for in situ monitoring. Adequate and sustained monitoring and its evaluation provides early warning of possible environmental degradation. Such information is important for the prediction of change that may be generated in the wake of a development project, such as dam construction or deforestation programs. In this context—as an element of an environmental impact assessment—monitored data are valuable for an evaluation of sustainability.
14. Explosives
All chemical explosives obtain their energy from the almost instantaneous transformation from an inherently unstable chemical compound into more stable molecules. The breakthrough from the 2000- year old ‘‘black powder’’ to the high explosive of today was achieved with the discovery of the molecular explosive nitroglycerine, produced by nitrating glycerin with a mixture of strong nitric and sulfuric acids. Nitroglycerin, because of its extreme sensitivity and instability, remained a laboratory curiosity until Alfred Nobel solved the problem of how to safely and reliably initiate it with the discovery of the detonator in 1863, a discovery that has been hailed as key to both the principle and practice of explosives. Apart from the detonator, Nobel’s major contribution was the invention of dynamite in 1865. This invention tamed nitroglycerine by simply mixing it with an absorbent material called kieselguhr (diatomous earth) as 75 percent nitroglycerin and 25 percent kieselguhr. These two inventions were the basis for the twentieth century explosives industry. Explosives are ideally suited to provide high energy in airless conditions. For that reason explosives have played and will continue to play a vital role in the exploration of space.
15. Feedstocks
The word feedstock refers to the raw material consumed by the organic chemical industry. Sometimes, feedstock is given a more restricted meaning than raw material and thus applied to naphtha or ethylene, but not petroleum. The inorganic chemical industry also consumes raw materials, but the feedstock tends to be specific to the process in question, such as sulfur in the case of sulfuric acid. The development and growth of new feedstocks has driven the evolution of the organic chemical industry over the last two centuries. To a large extent, the history of this industry is the history of its feedstocks. Until the nineteenth century, the only significant raw material for the nascent organic chemical industry was fermentation- based ethanol (ethyl alcohol). Gradually, the products of wood distillation also became important, only to be overshadowed after 1860 by the coal-tar industry. As the organic chemical industry expanded in both size and scope between 1880 and 1930, the need for new feedstocks became urgent. The competition between coal and petroleum was resolved in favor of the latter in the late 1950s. The petrochemical industry has been phenomenally successful, underwriting the postwar boom in organic chemicals and plastics and weathering the oil crises of the 1970s with minimal damage. Its long-term sustainability remains an issue, and increasing attention is being paid to renewable feedstocks.
16. Green Chemistry
The term ‘‘green chemistry,’’ coined in 1991 by Paul T. Anastas, is defined as ‘‘the design of chemical products and processes that reduce or eliminate the use and generation of hazardous substances.’’ This voluntary, nonregulatory approach to the protection of human health and the environment was a significant departure from the traditional methods previously used. While historically people tried to minimize exposure to chemicals, green chemistry emphasizes the design and creation of chemicals so that they do not possess intrinsic hazard. Within the definition of green chemistry, the word chemical refers to all materials and matter. Therefore, the application of green chemistry can affect all types of products, as well as the processes to make or use these products. Green chemistry has been applied to a wide range of industrial and consumer goods, including paints and dyes, fertilizers, pesticides, plastics, medicines, electronics, dry cleaning, energy generation, and water purification.
17. Industrial Gases
While the gases that are now commonly referred to as ‘‘industrial,’’ namely oxygen, hydrogen, carbon dioxide, and nitrogen, were not fully understood until the nineteenth century, scientists in the twentieth century moved rapidly to utilize the knowledge. Driven largely by the demands of manufacturing industries in North America and Western Europe, rapid improvements in the technology of production and storage of industrial gases drove what has become a multibillion dollar business, valued at $34 billion in 2000. At the start of the twenty-first century, industrial gases underpin nearly every aspect of the global economy, from agriculture, welding, metal manufacturing and processing, refrigerants, enhanced oil recovery, food and beverage processing, electronic component manufacturing, to rocket propulsion. Oxygen for metal manufacturing is the largest volume market, with chemical processing and electronics using significant volumes of hydrogen and lower volumes of specialty gases such as argon. One of the byproducts of the expansive use of industrial gas is an increase in undesirable environmental pollutants—contributing to the ‘‘greenhouse effect’’ and an overabundance of nitrates from agriculture application. Subsequently, government controls worldwide have led the gas industry to revamp some of its distribution and application. Hydrogen is likely to be the gas of the future, employed in ‘‘green’’ fuel cell technology, and glass and steel manufacturers are reducing nitrous dioxide emissions by mixing oxygen with coal.
18. Isotopic Analysis
Beyond the analysis of the chemical elements in a sample of matter, it is possible to determine the isotopic content of the individual chemical elements. The chemical analysis of a substance generally takes its isotopic composition to be a ‘‘standard’’ that represents terrestrial composition, because for most purposes the isotopic ratios are more or less fixed, allowing chemical weight to be a useful laboratory parameter for most elements. Deviations from the standard composition occur because of differences in: 1. Nuclear synthesis 2. Radioactive decay 3. Geological, biological, and artificial fractionation 4. Exposure to various sources of radiation Applications of isotopic analysis make use of these sources of variation. Our knowledge results from analytical techniques developed during the twentieth century that allow precisions of 10–5 and that have led to significant improvements in the understanding of the Earth and solar system and even of archaeology.
19. Nitrogen Fixation
In 1898, the British scientist William Crookes in his presidential address to the British Association for the Advancement of Science warned of an impending fertilizer crisis. The answer lay in the fixation of atmospheric nitrogen. Around 1900, industrial fixation with calcium carbide to produce cyanamide, the process of the German chemists Nikodemus Caro and Adolf Frank, was introduced. This process relied on inexpensive hydroelectricity, which is why the American Cyanamid Company was set up at Ontario, Canada, in 1907 to exploit the power of Niagara Falls. Electrochemical fixing of nitrogen as its monoxide was first realized in Norway, with the electric arc process of Kristian Birkeland and Samuel Eyde in 1903. The nitrogen monoxide formed nitrogen dioxide, which reacted with water to give nitric acid, which was then converted into the fertilizer calcium nitrate. The yield was low, and as with the Caro–Frank process, the method could be worked commercially only because of the availability of hydroelectricity.
In Germany, BASF of Ludwigshafen was interested in diversification into nitrogen fixation. From 1908, the company funded research into nitrogen fixation by Fritz Haber at the Karlsruhe Technische Hochschule. Haber specialized in the physical chemistry of gas reactions and drew on earlier studies started in 1903 on the catalytic formation of ammonia from its elements, nitrogen and hydrogen. He attacked the problem with high pressures, catalysts, and elevated temperatures. Even under optimum conditions the yield was low, around 5 percent, but Haber arranged for unreacted hydrogen and nitrogen to be recirculated. Though exothermic, the reaction was carried out at 600C in order to increase the rate. The preferred catalyst was either osmium or uranium. The main part of the apparatus was the furnace (later known as a converter) in which the gases were preheated by the outgoing reaction mixture. At a pressure of 200 atmospheres the gases were forced to react in the presence of the catalyst. Cooling moved the equilibrium in the direction of producing ammonia, which was liquefied and separated from unreacted hydrogen and nitrogen.
20. Oil from Coal Process
The twentieth-century coal-to-petroleum or synthetic fuel industry evolved in three stages: 1. Invention and early development of Bergius coal liquefaction (hydrogenation) and Fischer–Tropsch (F–T) gas synthesis from 1910 to 1926. 2. Germany’s industrialization of the Bergius and F–T processes from 1927 to 1945. 3. Global transfer of the German technology to Britain, France, Japan, Canada, the U.S., and other nations from the 1930s to the 1990s. Petroleum had become essential to the economies of industrialized nations by the 1920s. The mass production of automobiles, the introduction of airplanes and petroleum-powered ships, and the recognition of petroleum’s high energy content compared to wood and coal, required a shift from solid to liquid fuels as a major energy source. Industrialized nations responded in different ways. Germany, Britain, Canada, France, Japan, Italy, and other nations, having little or no domestic petroleum, continued to import it. Germany, Japan, and Italy also acquired by force the petroleum resources of other nations during their 1930s–1940s World War II occupations in Europe and the Far East. In addition to sources of naturally occurring petroleum, Germany, Britain, France, and Canada in the 1920s–1940s synthesized petroleum from their domestic coal or bitumen resources, and during the 1930s–1940s war years Germany and Japan synthesized petroleum from the coal resources they seized from occupied nations. A much more favorable energy situation existed in the U.S., and it experienced few problems in making an energy shift from solid to liquid fuels because it possessed large resources of both petroleum and coal.
21. Radioactive Dating
There are natural radioactive isotopes that have half-lives comparable to the age of the earth, the most familiar being uranium-235 and -238(235U, 238U) and thorium-232 (232Th). The decay of these isotopes proceeds sequentially through intermediate products having much shorter half-lives to the stable isotopes of lead, 207Pb, 206Pb, and 208Pb, respectively. There are 14 other isotopes scattered through the periodic table that have half-lives spanning times from 1015 to 109 years. All can be used for dating geologic materials. There are other isotopes, termed cosmogenic isotopes, that are produced by cosmic rays and that have half-lives that are useful for measuring periods of historical interest. The best known of these is carbon-14 (14C), which has important uses in archaeology because of its half-life of 5760 years. Procedures for measuring isotope composition have the disadvantage of destroying the extracted portion of the sample used. The phenomena that allow nondestructive analysis rely on exciting optical radiation or x-radiation from the atoms of the sample, most commonly induced by electron bombardment. In these methods, isotopic effects are too small to be accurately observed.
22. Reppe Chemistry
Reppe chemistry refers to a group of high-pressure reactions used in industry to make various organic chemicals. Most of these reactions were based on acetylene, and as acetylene declined in popularity (because of the cheapness of ethylene) in the 1960s, most of the Reppe reactions have also lost their significance. However, the formation of butanediol from acetylene has survived and is now one of the few acetylene-based processes used in the chemical industry. Walter Reppe who was working for the German firm of IG Farben at Ludwigshafen on the Rhine, discovered in 1932 that alcohols could be added to acetylene under considerable pressure to form the corresponding vinyl ether. The dangers of using acetylene were well known, and the success of this reaction was unexpected. The vinyl ethers were used as the starting point for the production of polyvinyl ethers, which were considered to be possible alternatives to polyvinyl chloride (PVC). Subsequently they turned out to have only limited applications, for instance, as a synthetic substitute for chewing gum.
23. Solvents
Solvents are the hidden element in a broad range of technological activities, including chemical processes, paint, dry cleaning, and metal degreasing. They are used to dissolve organic compounds (water is the usual solvent for inorganic compounds) to enable reactions or polymerization, spreading or ease of use, or to extract compounds from a matrix such as plant material. Dry cleaning is a specialized form of the last group, as it removes fatty substances that adhere dirt to clothing. Many organic compounds either react with or do not dissolve in water, hence the need to find suitable organic chemicals to act as the solvent. It is hard to find compounds that dissolve a wide range of substances, but are also relatively cheap, nonflammable and nontoxic. In practice, the solvents used represent a compromise, often an unsatisfactory one.
The first solvent to be readily available was ethanol (ethyl alcohol) made by fermentation and distillation since the late Middle Ages. Amyl alcohol (fusel oil), a byproduct of ethanol manufacture, also became a popular solvent. Oil of turpentine, made from pine resin, became an important solvent in the eighteenth century and was used in paint and varnishes, and to dissolve rubber. It was also the basis of the earliest form of dry cleaning, which started around 1825. Wood spirit, made by dry distilling wood, first appeared in the early nineteenth century, but its purification into methanol (wood alcohol, methyl alcohol), acetone, and methyl ethyl ketone only took place in the middle of that century. As late as 1914, acetone and methanol were the only significant solvents in the U.S. (by volume), which had access to extensive virgin woodland. Synthetic methanol, made by treating carbon monoxide with hydrogen under pressure, was first made by the German chemical firm BASF in 1923 and eventually replaced the natural product.
24. Synthetic Resins
Chemistry became particularly conspicuous in the twentieth century through synthetic polymers. They include resinous products that are converted into plastics, laminates, surface coatings, and adhesives. Polymers exist because carbon has the property of forming single and multiple bonds with other carbons. In 1922 Hermann Staudinger suggested that polymers were macromolecules. Despite initial opposition, his ideas were accepted from around 1930 and had a considerable impact on industrial developments. The theory and mechanism of the processes whereby small molecules, the monomers, join in repeating units to create giant molecules, the polymers, was established around 1930, following the studies of Wallace Hume Carothers at the DuPont Company in the U.S. He identified two processes, condensation and addition, that distinguish between the main types of products. This provides a useful means for understanding historical developments.
From around 1900, chemists, electrical engineers, and inventors sought out novel products to replace or supplement natural rubber and gutta percha. Most promising was the chemical reaction between phenol and formaldehyde. Leo Hendrik Baekeland, a Belgian who had emigrated to the U.S., carefully controlled the conditions and recognized the catalytic action of acids and bases. He perfected the process in 1907. His main product was a resin readily converted into the first of the thermoplastics, those that set hard and rigid. Baekeland set up the General Bakelite Company in Perth Amboy, New Jersey, in 1910. Another early inventor was Sir James Swinburne, in England, but his process was covered by Baekeland’s patent.
25. Synthetic Rubber
Rubber is a ubiquitous material in modern society, enhancing the quality of life in a myriad of applications. It was unknown in the Western world until the Spanish began their explorations of America, where they found Indian tribes playing games with a ball made from the milky sap obtained by cutting the bark of local trees. In France this sap, or latex, was called caoutchouc after the native name for the ‘‘weeping tree’’ that produced it, while the English called it Indian rubber because it was useful for removing pencil marks from paper. A number of trees and shrubs produced such latex, including the orders Euphorbiaceae, Urticaceae, Apocynaceae, and Asclepiadaceae, but only two natural sources became commercially important (Hevea brasiliensis and Parthenium argentatum).
The use of rubber for practical purposes was slow to develop because the tree latex coagulated quickly and was difficult to process in the solid form. After solvents were discovered that would dissolve the solid rubber, products were made that took advantage of rubber’s elasticity and waterproofing capability, but these crude materials suffered from an inherent stickiness and a form that changed depending on the temperature. With the discovery of the vulcanization process by Charles Goodyear in 1839, the rubber industry had a technique for eliminating these difficulties and better consumer products soon appeared on the market.
After World War II, synthetic rubber plants were built worldwide, and by 1960 the use of synthetic rubber surpassed that of natural rubber for the first time. According to the International Institute of Synthetic Rubber Producers, by the end of 2001, ‘‘The yearly capacity of synthetic rubber manufacturing plants around the globe totals about 12 million metric tons and the capacity of tree-grown natural rubber produced on rubber plantations is approximately 8 million metric tons.’’
History of Chemical Industry and Technology

Although people have made and used chemicals for thousands of years, the modern industry, based on large-scale production, emerged during the Industrial Revolution of the late eighteenth and early nineteenth centuries. The first industrial chemical—sulfuric acid—dates from the mid-eighteenth century when large lead-lined chambers were used to allow the oxidation of sulfur dioxide, made by burning sulfur, to sulfur trioxide, which reacts with water to produce acid. By the mid nineteenth century sulfuric acid plants had grown very large and had reached a high degree of technical sophistication, incorporating most of the techniques of modern chemical engineering. The availability of cheap sulfuric acid allowed the development of cheap alkali by the LeBlanc process, first developed in France but commercialized in Great Britain after 1810. Sulfuric acid was converted to sodium carbonate through a series of reactions with salt, limestone, and charcoal. Large quantities of acids and bases were consumed in Great Britain principally in textile operations, such as washing, bleaching, and dyeing.
Armed with these two reagents—acid and base—chemists began to experiment with a wide variety of substances, many of them organic (carbon-containing). By midcentury chemists had discovered some useful new compounds. In 1856, a young English chemist, William H. Perkin while naively trying to convert coal-tar into the valuable antimalarial quinine produced a purple colored solution instead. At the moment of this discovery extremely expensive purple was the fashionable color among Europe’s elite. Using cheap coal-tar, a waste product from coal gasification plants that supplied illuminating gas to cities, Perkin developed a process to make a purple dye, mauve, by oxidizing aniline (benzene with an ammonia group substituted for a hydrogen atom). Other chemists soon discovered that the larger class of chemicals based on benzene rings would yield a rainbow of colors when reacted with acids and bases. The systematic and highly profitable exploitation of aniline dyes shifted in the 1870s to the new nation of Germany where the government, universities, and emerging chemical companies cooperated to develop this important industry. By World War I, three German companies, Bayer, BASF, and Hoechst controlled about 90 percent of the world’s dyestuffs production. German chemists isolated the chemicals made by the madder and indigo plants that produced red and blue dyes, respectively. Chemists and engineers then learned how to manufacture these chemicals from coal-tar chemicals, replacing natural dyes which were major agricultural products of several countries, especially Turkey (madder) and India (indigo). Dyestuffs chemistry led German chemists into new fields such as pharmaceuticals with the discovery of aspirin by Felix Hoffmann and salvarsan (the first effective treatment for syphilis) by Paul Erlich. Another dyestuffs-related chemical, TNT (trinitrotoluene) would play a critical role as a shell-bursting explosive in World War I.
Explosives were revolutionized by chemists beginning in the middle of the nineteenth century. Experiments with nitric acid and organic molecules resulted in nitrate groups bonding onto the organic molecules, creating highly flammable or even explosive compounds. This characteristic resulted from the molecular proximity of a fuel (the organic compound) and oxygen (there are three oxygen atoms in each nitrate group). The most notorious of these new compounds was nitroglycerin, a liquid with tremendous explosive energy that was so unstable it often detonated prematurely. In Sweden, Alfred Nobel stabilized nitroglycerin by absorbing it into diatomaceous earth to produce a putty-like substance that could be extruded into paper casings. He called his product dynamite, and beginning in the 1870s it displaced black powder in blasting operations. Dynamite was one of the technological advances that would make projects such as the Panama Canal feasible.
Even more important than dynamite was its chemical cousin, nitrocellulose, prepared by reacting nitric acid with cotton fibers. This still cotton-like material became the basis for smokeless powder, which in the 1890s began to replace black powder as the propellant in guns and cannon. Smokeless powder burned much more cleanly than black powder and was much more powerful. The new propellant made the machine guns and heavy artillery into the terribly effective weapons that turned World War I into a bloody stalemate. Smokeless powder had a tendency to decompose causing spontaneous fires and explosions, until German chemists discovered a dyestuffs- related compound that stabilized the powder in 1908. Another key chemical in the munitions machine was TNT, which exploded on shell impact causing huge craters and saturating the air with shrapnel.
The ingenuity of chemists added another horrific element to life in the trenches—poison gas. At the Battle of Ypres in 1915, German chemist Fritz Haber orchestrated the release of 5000 cylinders of chlorine which drifted with the wind into the Allied lines. The burning, choking gas caused panic in the Allied army but the Germans were not prepared to attack and so lost the advantage of its new weapon. Afterward both sides used poison gases such as lewisite, phosgene and mustard gas throughout the remainder of the war. All of these gases contained chlorine, which could be made in large quantities using electrochemical technology.
The development of the dynamo in the 1870s made available large quantities of electricity that could be used to make chemicals, many of which could not be economically made by other methods. Perhaps, the most important example was aluminum, which had semiprecious metal status—a small pyramid of it capped the Washington Monument which was completed in 1883. Three years later, Charles Martin Hall in the U. S. and Paul Louis Toussaint Heroult in France discovered a process to make aluminum using electricity. This method is still used today.
Another important electrochemical process was the production of chlorine and caustic soda (sodium hydroxide) from salt water. Chorine was used principally in bleaching powder and sodium hydroxide became the major base, replacing earlier compounds such as sodium carbonate. This process began to be used in 1890s; several electrolytic plants were built near Niagara Falls where hydroelectric power was available, and Herbert Dow built an early plant in Midland, Michigan where there was a rich supply of brine wells.
Other important materials were made in electric furnaces, which could generate very high temperatures, invented by Henry Moissan in 1892. One new ceramic compound was silicon carbide, which is so hard that it can be used to shape metals by grinding. Another was calcium carbide which reacts with water to produce acetylene, used in early automobile head lights and in oxyacetylene metal-cutting torches. Made from coal, acetylene became an early chemical building block used to make other chemicals.
The development of the Haber–Bosch ammonia process between 1906 and 1912 was a technological and scientific tour de force that became a prototype for future chemical processes. One of the great scientific and technological challenges of the late nineteenth century was ‘‘fixing nitrogen.’’ Nitrogen was an essential ingredient in explosives and fertilizer. Most of the world’s useable nitrogen came from nitrate mines in the Atacama desert in northern Chile. Of course, air is 80 percent nitrogen, but it is almost chemically inert because it consists of two tightly bound atoms. Chemists sought ways to break those bonds. One way to do this was to react nitrogen and hydrogen to make ammonia. On paper it looked simple; in the laboratory it did not happen under normal conditions. A solution to this apparent impasse was suggested by theoretical considerations derived from the evolving disciplines of kinetics (the rate of chemical reactions) and chemical thermodynamics (determines the feasibility of particular reactions). The ammonia reaction was found to be feasible by German chemists Walter Nernst and Fritz Haber. Their calculations showed that the reaction would occur at very high temperatures (for kinetics) and very high pressures (for thermodynamics). The challenge then became technological: was it possible to build steel vessels that could withstand temperatures of 500C and a pressure of 200 atmospheres? After Haber was able to make ammonia in laboratory scale apparatus, Carl Bosch of the BASF Company oversaw the development of a commercial process. Some of the early reactors were made from Krupp cannons. An essential part of the process was the development of a catalyst, a substance that causes the nitrogen and hydrogen to react with each other. At BASF, Alwin Mittasch led an exhaustive search until an efficient and durable iron-based catalyst was developed. The first large plant started up in 1913 a year before World War I would make Chilean nitrates unobtainable in Germany because of Britain’s dominance of the seas. Without ‘‘synthetic’’ nitrogen, the Germans could not have sustained their war effort for four years.
In the 1920s BASF would expand on its high-temperature, high-pressure technological base by developing processes to make methanol from carbon monoxide and hydrogen and gasoline from coal. Before the new process, methanol was obtained by distilling it from wood (hence its name wood alcohol). The synthetic gasoline project was initiated by predictions of impending shortages of crude oil. After 1929, the discovery of the east Texas oil field increases world crude supplies and the Great Depression lowered demand for gasoline, the huge investment in synthetic gasoline technology threatened the viability of the giant IG Farben chemical combine. (The major German chemical companies had merged in 1925 primarily to sustain export markets.) The project and company would be rescued by Hitler after he came to power in 1933, since a domestic supply of gasoline—Germany has no oil—would be essential in a future war.
Hitler’s policy of autarky sustained another project that would have important consequences for the chemical industry—synthetic rubber. Making synthetic versions of natural materials had been a long-standing objective of the chemists and one of the foundations of the chemical industry. Dyestuffs had been the first major success, but chemists also sought to make other substances, especially silk and rubber. Until the 1920s the basic structure of these substances was a matter of scientific uncertainty. This, however, did not stop chemists from forging ahead trying to make synthetic substitutes for exotic and expensive natural materials.
The origin of synthetic materials dates to 1870 when Albany tinkerer, John Wesley Hyatt formed a solid plastic from a mixture of nitrocellulose and camphor, which he called celluloid. According to tradition, Hyatt was looking for a substitute for expensive elephant ivory in billiard balls. When his new material failed in this use, he then made celluloid look like exotic materials—ivory, amber, and tortoiseshell—so it would be used in toilet sets, toys, and numerous other trinket-like applications. Its most enduring legacy was as the film base that made motion pictures possible beginning in the 1890s. An unsuccessful use of nitrocellulose was as an artificial silk fiber that, among other deficiencies, was highly flammable.
A much better silk-like fiber was rayon, formed by dissolving cellulose to make a syrupy viscose solution that was extruded through small holes in a plate into another chemical bath that solidified the fiber. Charles Cross and Edward Bevan in Britain discovered this process in the 1890s, while attempting to make improved light bulb filaments. After 1910 the market for rayon fibers began to expand rapidly worldwide; the fashion industry embraced it the 1920s; and during the Great Depression it replaced silk in all apparel except stockings. Rayon was the biggest new product for the chemical industry in the interwar years.
Rayon was just one a growing number of products made of large molecules (or macromolecules), in this case it was natural cellulose. Chemists were beginning to make entirely new large molecules. A pioneer is this effort was Leo Baekeland who invented a hard plastic he dubbed Bakelite in 1907. The new material was made by heating phenol and formaldehyde under pressure. Among the many uses for Bakelite was as a substitute for ivory in billiard balls.
The growing importance of and interest in large molecules in the 1920s sparked a scientific debate, especially in Germany—still the center of chemistry— about their structure. Although many chemists argued that large molecules were held together by peculiar forces, Hermann Staudinger put forth the hypothesis that large organic molecules were just that—larger versions of common organic chemicals held together by same types of chemical bonds. Following Staudinger, Wallace H. Carothers, a researcher in the DuPont Company developed methods for making large molecules, or polymers, in the laboratory. Out of this research DuPont researchers discovered neoprene synthetic rubber (1930) and nylon (1934). By 1940 neoprene had established itself as a specialty rubber and nylon had become the preferred stocking fiber. Once the mysteries surrounding polymers had been solved, chemists everywhere began to explore this large and promising new field.
Perhaps the most significant discovery, both historically and for the future chemical industry, was made in 1929 by IG Farben chemists who made a general purpose synthetic rubber from a polymer consisting of repeating units of butadiene and styrene. At the time of this breakthrough virtually all of the world’s rubber came from British controlled plantations in Malaysia. By early 1942, these were all in Japanese occupied territory. The first year of American fighting was hampered by a lack of rubber which threatened to bring the effort to a thudding halt. To resolve this crisis the U.S. government organized a cooperative venture between oil, chemical, and tire companies to rapidly build up an American synthetic rubber capability. This initiative was a marked success, production went from nothing to 800,000 tons in two years. One of the major obstacles that had to be overcome was to develop processes to make enormous quantities of styrene and butadiene. Styrene was available before the war in limited quantities but butadiene was not a commercial chemical. The supply of butadiene came primarily from oil companies, which had previously concentrated on making fuels not chemicals.
In the interwar years a few companies such as Union Carbide and Shell Oil had begun to make chemicals from petroleum and natural gas. One notable product introduced in the 1930s was ethylene glycol—automobile radiator coolant antifreeze. Until World War II organic chemicals used as feedstocks for the chemical industry were distilled from coal. For example, the type of nylon DuPont commercialized was determined mainly by the abundance of benzene, a major coal impurity. After World War II the oil and chemical industries, especially in the U.S., would soon shift to petrochemical feedstocks.
The oil industry was now generating large quantities of chemicals as a byproduct of new processes developed to produce more gasoline from a barrel of crude of oil and to produce higher octane fuels, especially necessary for aviation gasoline during World War II. Crude oil is a complex mixture of hydrocarbons with varying carbon atom chain lengths. Originally, natural gasoline, which contains five to nine carbons in each molecule was distilled out of crude oil. In 1913, E. M. Burton developed a cracking process in which he subjected the heavier fractions of crude oil to heat and pressure which broke the larger molecules into smaller ones, some of which were in the gasoline-size range. In the 1930s, French inventor and engineer, Eugene Houdry, added a catalyst to this process which significantly improved its overall performance. A decade later, an improved catalytic process called fluidized bed cracking was developed by Massachusetts Institute of Technology chemical engineers and Standard Oil of New Jersey. This process has been used ever since. Also during the late 1930s oil companies began to develop processes to combine some of the smaller cracked molecules into larger ones that could boost the octane rating of gasoline. During World War II, American 100-octane aviation fuel helped Allied pilots win the air war over Europe.
A few years after the war ended, the Universal Oil Products company, a research organization, introduced a new process which had been developed by Vladimir Haensel. Called ‘‘platforming’’ because of its platinum catalyst, it dramatically improved the octane rating of gasoline primarily by stripping hydrogen atoms from cyclical compounds, converting them to benzene, tolulene, and xylene. These compounds were not only important for high-octane gasoline but were in great demand by the chemical industry as raw materials, especially for the booming plastics and polymers businesses.
The dramatic post-World War II expansion of the chemical industry was led by plastics and polymers. Shortages of metals and other materials during the war had prompted the U.S. government to encourage manufacturers to use plastics for a wide variety of applications. For example, vinyl resin (mostly polyvinyl chloride or PVC) production increased from 2.3 million to 100 million kilograms. Although many plastics ended up in applications such as army bugles, others served essential high-technology functions. Polyethylene, a difficult to make plastic, had unique insulating properties needed for radar. DuPont’s exotic polymer Teflon, which did not melt, dissolve in solvents, or stick to anything, was used as a sealant in the Manhattan Project for the atomic bomb. Clear acrylic plastic became the material for airplane windows and bomber gunner turrets.
After the war both the uses and varieties of polymers increased to fulfill the demand by consumers for whom convenience became a hallmark of modern life. A New England inventor, Earl Tupper, introduced his line of polyethylene food storage containers—Tupperware—that preserved leftovers and kept them neatly in the refrigerator. The most sensational new products were synthetic textile fibers that made clothing more affordable, machine washable, drip dry, and wrinkle free. DuPont’s nylon took over the stocking market and made major inroads in other apparel. Polyester, discovered by two British chemists in 1940, was used instead of wool in suits and blended with cotton to make permanent press garments. Acrylic fibers made sweaters, especially lightweight ones, popular with postwar women. By 1956, synthetic fibers had eclipsed wool as the number two textile fiber consumed in the U.S. By 1970, synthetic fiber consumption surpassed that of cotton in apparel.
At the same time that synthetic fibers were revolutionizing modern wardrobes, new types of plastics found myriad uses. The major breakthrough in plastics was made when German chemist, Karl Ziegler in 1953 discovered a new type of catalyst that produced new kinds of polymers, notably linear polyethylene and polypropylene. These two plastics had outstanding properties such as toughness which led to many uses, especially food packaging. As polymer science matured in the 1950s, chemists made more complex and sophisticated compounds, examples being DuPont’s Kevlar polyaramid and Lycra spandex fibers.
Another significant growth sector for the post- World War II chemical industry was organic-chemical based pesticides. The archetype was DDT, whose remarkable kill-on-contact property was discovered by Paul Mueller in Switzerland in 1939. Most earlier insecticides were poisons, such as lead arsenate, that had to be ingested. During the war, George W. Merck, working on biological warfare for the government, discovered that a DuPont plant growth compound called 2,4-D was actually an effective herbicide. After the war chemical companies focused research efforts on finding new insecticides, herbicides, and fungicides. Although Rachel Carson’s Silent Spring (1962) publicized the toxic effects of DDT on birds and raised questions about the effect of pesticides on human health, during that decade 96 new insecticides, 110 new herbicides, and 50 new fungicides were introduced. Insecticides included organophosphorus compounds, carbamates, and synthetic pyrethrins. DuPont in 1967 introduced Benlate (benomyl), the first fungicide that was taken up internally rather than being effective only on the leaf surfaces. In the 1970s Monsanto introduced its blockbuster herbicide, Roundup (glyphosate), which was suitable for a wide variety of crops. In the 1990s chemical companies, especially Monsanto and DuPont combined biotechnology and herbicides to create crop seeds that were compatible with specific herbicides, and to incorporate insecticidal properties into plants by splicing in genes from other organisms. These so-called genetically modified foods have created controversy in Europe but have met with little resistance in the U.S.
It became evident in the 1960s that the chemical industry was maturing. During the 1970s the industry was beleaguered by spikes in the cost of energy and feedstocks, and environmental legislation that required major capital investments in pollution control and abatement. By the 1980s, the chemical industry had become very competitive worldwide with growth and profits tightly linked to larger business cycles. Since then the industry had undergone massive reorganization in response to these new economic realities. For the most part, chemicals, if not the companies that make them, have become commodities. Because it still has significant research capabilities, the chemical industry is hoping that new technologies such as nanotechnology—very small molecular structures— or green chemistry—replacing petroleum with renewable feedstocks—might restore chemicals to the essential status it enjoyed in the twentieth century.
ORDER HIGH QUALITY CUSTOM PAPER

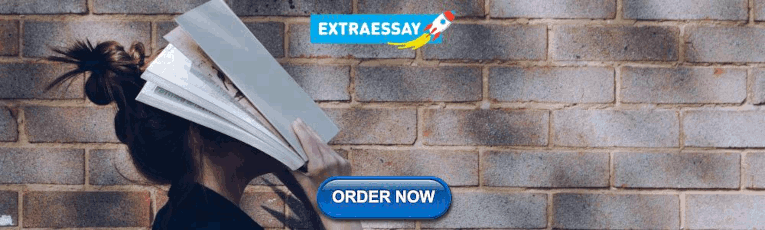
IMAGES
VIDEO
COMMENTS
Nov 2022. Explore the latest full-text research PDFs, articles, conference papers, preprints and more on CHEMICAL TECHNOLOGY. Find methods information, sources, references or conduct a literature ...
Within the Chemical Engineering Journal, the Chemical Reaction Engineering section presents papers on a wide range of topics including reaction kinetics, simulation and optimization of different types of reactors, unsteady-state reactors, multiphase reactors, and process intensification including fundamental investigations of the processes of ...
Chemical engineering is a branch of engineering that deals with the processes (production, transformation, transportation and usage) necessary to produce useful materials and energy. Chemical ...
The Journal of Chemical Physics is an international journal that publishes cutting edge research in all areas of modern physical chemistry and chemical physics. The Journal also publishes brief communications of significant new findings, perspectives on the latest advances in the field, and Special Topics. Read more about the journal
The Journal of Environmental Chemical Engineering - JECE provides a forum for the publication of original and innovative research on the development of advanced, safer, green and sustainable environmental technologies towards a carbon-neutral circular and self-sufficient bio-based economy, focusing on soil, water, wastewater and air decontamination; pollution monitoring, prevention and control ...
Separation and Purification Technology is a journal dedicated to the dissemination of novel methods for separation and purification in chemical and environmental engineering for homogeneous solutions and heterogeneous mixtures. This includes any separation and/or purification of liquids, vapors and gases, carbon capture and separation, with the exception of methods intended for analytical ...
Sensors & Actuators, B: Chemical is an international journal devoted to research and development of chemical transducers. It is an interdisciplinary journal dedicated to publishing research and development in the field of chemical sensors and biosensors, chemical actuators and analytical … View full aims & scope $
High quality research in physical chemistry, chemical physics and biophysical chemistry. Editorial Board Chair: Anouk Rijs Impact factor: 2.9 Time to first decision (peer reviewed only): 34 days ... Journal of the Chemical Society, Faraday Transactions 1: Physical Chemistry in Condensed Phases (1972-1989) Journal of the Chemical Society ...
Formerly: Journal of Mechanical Working Technology. The Journal of Materials Processing Technology covers the processing techniques used in manufacturing components from metals and other materials. The journal aims to publish full research papers of original, significant and rigorous work and so to contribute to increased production efficiency and improved component performance.
Chemistry articles from across Nature Portfolio. Chemistry is a branch of science that involves the study of the composition, structure and properties of matter. Often known as the central science ...
A University of Virginia-led research team has developed new protective coatings that allow turbine engines to run at higher temperatures before components begin to fail. ... a chemical reaction that occurs with exposure to air and moisture. However, these systems are limited by the melting temperature of one layer, silicon, which melts at ...
The Department of Applied Biology and Chemical Technology at the Hong Kong Polytechnic University (PolyU) has a long history of interdisciplinary research in chemistry and biology. From 2001 to 2009, with the strong support of the Hong Kong Research Grants Council, the Institute of Molecular Technology for Drug Discovery and Synthesis was ...
Institute of Chemical Technology (ICT) is a public deemed university in Mumbai, India.It is focused on training and research in the fields of chemical engineering, chemical technology, and pharmaceutical sciences.Established in 1933, the institute was granted deemed university status in 2008, making it the only state-funded deemed university in India. . In 2018, ICT was named an institute with ...
The International Journal of Scientific & Engineering Research is a one-stop, open access source for a large number of high quality and peer reviewed journals in all the fields of science, engineering and technology. Scientists and engineers involved in research can make the most of this growing global forum to publish papers covering their original research or extended versions of already ...
Department of Chemical Engineering 77 Massachusetts Avenue, Room 66-350 Cambridge, Massachusetts 02139
An international journal devoted to the science and application of advanced surface treatments for improvement of material properties. Surface and Coatings Technology is an international archival journal publishing scientific papers on significant developments in surface and interface engineering to modify and improve the surface properties of materials for protection in demanding contact ...
No matter what, always get your research paper topic approved by your teacher first before you begin writing. 113 Good Research Paper Topics. Below are 113 good research topics to help you get you started on your paper. We've organized them into ten categories to make it easier to find the type of research paper topics you're looking for. Arts ...
This collection of chemical technology research paper topics provides the list of 25 potential topics for research papers and an overview article about history of chemical industry and technology.. 1. Biopolymers. Biopolymers are natural polymers, long-chained molecules (macromolecules) consisting mostly of a repeated composition of building blocks or monomers that are formed and utilized by ...
Key Takeaways. Today, we're publishing our research paper that dives into the underlying technology powering Stable Diffusion 3.. Stable Diffusion 3 outperforms state-of-the-art text-to-image generation systems such as DALL·E 3, Midjourney v6, and Ideogram v1 in typography and prompt adherence, based on human preference evaluations.
Journal of Engineering Research (JER) is an international, peer reviewed journal which publishes full length original research papers, reviews and case studies related to all areas of Engineering such as: Civil, Mechanical, Industrial, Electrical, Computer, Chemical, Petroleum, Aerospace, Architectural, etc. JER is intended to serve a wide range of educationists, scientists, specialists ...