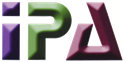
- Pompe Registry
- Patient-Focused Publications
- Patients Helping Patients
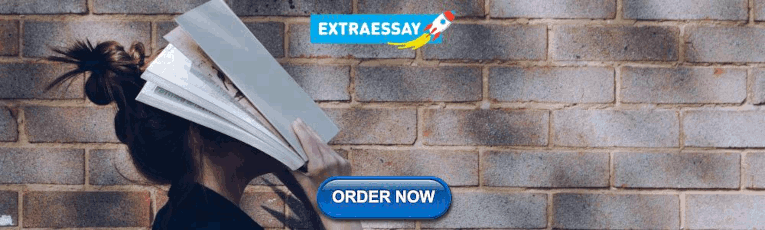
Research Papers
- Publications for Physicians
- Testimonials
- International Pompe Day
- IPA Erasmus Pompe Survey
- IPA Members & Contacts
- IPA Community Advisory Board
- IPA Reviews & Reports
- IPA Conferences
- IPA Position Papers
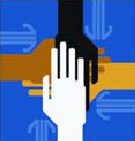
A research paper is a document that presents the findings of a scientific study or research project. It is written by researchers who conduct experiments or investigations to explore a specific topic or question. The paper follows a specific format, including sections like introduction, methods, results, discussion, and conclusion.
Researchers use research papers to share what they have learned about a particular subject. These papers go through a rigorous peer review process to ensure their quality. Research papers contribute to the collective knowledge in a field, help professionals stay updated with advancements, and influence healthcare practices and policies. While they may be challenging to understand without a scientific background, discussing research findings with your healthcare provider can help you understand their relevance to your specific situation and guide your treatment options.
2015 Research Papers

2014 Research Papers
2013 research papers.
- Impact of enzyme replacement therapy on survival in adults with Pompe disease: results from a prospective international observational study
- Phase I/II Trial of Adeno-Associated Virus–Mediated Alpha-Glucosidase Gene Therapy to the Diaphragm for Chronic Respiratory Failure in Pompe Disease: Initial Safety and Ventilatory Outcomes (ABSTRACT ONLY)
2012 Research Papers
- Effect of enzyme therapy and prognostic factors in 69 adults with Pompe disease: an open-label single-center study
Recent Articles
- Amicus Therapeutics Announces Approval and Launch of New Pompe Disease Therapy in the European Union
- Patient Registries for Pompe Disease Survey
- 2023: Every Move Counts
- Rare Disease Day: 29 February 2024
- International Pompe Day: 15 April 2023
- International Pompe Day: 15 April 2024 – Every Move Counts
Pompe Disease: From Basic Science to Therapy
- Published: 16 August 2018
- Volume 15 , pages 928–942, ( 2018 )
Cite this article
- Lara Kohler 1 ,
- Rosa Puertollano 1 &
- Nina Raben ORCID: orcid.org/0000-0001-9519-3535 1
15k Accesses
118 Citations
10 Altmetric
Explore all metrics
Pompe disease is a rare and deadly muscle disorder. As a clinical entity, the disease has been known for over 75 years. While an optimist might be excited about the advances made during this time, a pessimist would note that we have yet to find a cure. However, both sides would agree that many findings in basic science—such as the Nobel prize-winning discoveries of glycogen metabolism, the lysosome, and autophagy—have become the foundation of our understanding of Pompe disease. The disease is a glycogen storage disorder, a lysosomal disorder, and an autophagic myopathy. In this review, we will discuss how these past discoveries have guided Pompe research and impacted recent therapeutic developments.
Similar content being viewed by others
Diagnostic Approach to Proximal Myopathy
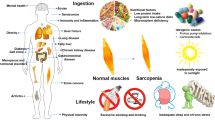
Advances in sarcopenia: mechanisms, therapeutic targets, and intervention strategies
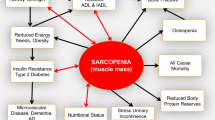
Sarcopenia: no consensus, no diagnostic criteria, and no approved indication—How did we get here?
Avoid common mistakes on your manuscript.
Background and History
Pompe disease, a severe metabolic myopathy, is caused by mutations in the gene coding for acid alpha-glucosidase (GAA), the enzyme that breaks down glycogen in acidic milieu of the lysosome. Once in the lysosome, glycogen can escape following complete degradation by GAA in the form of glucose. A deficiency of the enzyme leads to lysosomal accumulation of glycogen in multiple tissues, but cardiac and skeletal muscles are most severely affected. The disease also goes by the name “Type II glycogen storage disease (GSDII)” or “Acid maltase deficiency.” It is named after a Dutch pathologist, Johannes Cassianus Pompe, who described an autopsy of a 7-month-old girl diagnosed with “idiopathic myocardial hypertrophy” and generalized muscle weakness [ 1 ]. Dr. Pompe provided an insight into the underlying biology of the disease—massive vacuolar glycogen storage in virtually all tissues. The same year, 1932, similar cases were described [ 2 , 3 ].
Decades later, basic science breakthroughs led to the discovery of the metabolic pathway of glycogen [ 4 ] and a new cellular organelle, the lysosome, a ubiquitous membrane-bound vesicle which contains hydrolytic enzymes and an acidic intraluminal pH [ 5 ]. The glycogen-degrading enzyme, acid alpha-glucosidase, that normally resides in the lysosome and is missing in Pompe disease was discovered by a Belgian biochemist Henri-Gery Hers in 1963 [ 6 ]. Furthermore, Dr. Hers predicted that “other deposition diseases might be explained on the basis of the absence of other lysosomal enzymes,” thus triggering the search for enzymes responsible for the storage compounds in other lysosomal storage diseases (LSDs). Pompe disease has the distinction of being the first documented lysosomal storage disease; there are now more than 60 such disorders.
Biosynthesis of Acid Alpha-Glucosidase and Genetic Defects
The enzyme is synthesized as a 110 kDa precursor, which undergoes extensive posttranslational modifications in the rough endoplasmic reticulum (ER) on the way to the lysosomes; the activity of the enzyme is increased during the process [ 7 ]. The glycosylation and proper folding in the ER are crucial for transport to the Golgi where the enzyme acquires a mannose 6-phosphate (M6P) lysosomal targeting signal. The phosphorylation through the addition of M6P groups is a prerequisite for binding of the enzyme to the mannose 6-phosphate receptor (M6PR). The M6PR-captured enzyme is contained in a vesicle that pinches off from the Golgi to endosomes, where the enzyme dissociates from the receptors. The enzyme is then delivered to the lysosome, whereas the receptors cycle back for the next round of sorting, with some diverging and ending up at the plasma membrane. On the way to the lysosome, the enzyme is proteolytically cleaved at both the amino- and carboxyl-termini, a process critical for catalytic activation of the enzyme [ 7 , 8 , 9 , 10 ].
Pompe disease presents as a continuum of clinical phenotypes that differ by age of onset, severity, and organ involvement. The clinical course is largely dependent on the specific mutation and the resulting level of residual GAA activity, although genetic background and modifying factors may play a role [ 11 ]. A potential role of angiotensin-converting enzyme polymorphism in modulating the clinical outcome was shown in a group of patients with the late-onset form of the disease [ 12 ]. Hundreds of mutations have been described in the GAA gene on chromosome 17 q25 [ 13 , 14 , 15 ]. A database containing all the reported mutations and polymorphisms and information about their severity can be found on the homepage of the Pompe Center at Erasmus University in Rotterdam: www.pompecenter.nl .
Most mutations are found in a single family or a small population, and most patients are compound heterozygotes. The mutations are spread throughout the gene and affect different steps involved in the complex process of generating fully functional GAA including protein synthesis, posttranslational modifications, and lysosomal trafficking and maturation. Several mutations are commonly found in patients of certain ethnic backgrounds. Among these, c.-32-13T>G (IVS1) is the most common defect in Caucasians; this leaky mutation allows for the generation of low levels of normal enzyme [ 16 , 17 , 18 ]. Most often, this mutation is found in compound heterozygotes in combination with a second far more severe GAA mutation. Individuals homozygous for the IVS1 mutation were not expected to show any symptoms, but this long-standing assumption turned out to be incorrect. A recent report described myalgia, exercise-induced fatigue, and an increase in creatine kinase (CK), a marker of muscle damage, in patients harboring two IVS1 mutations [ 19 ] (see also section “ Newborn Screening ”).
c.del525, del exon18, and c.925G>A (p.Gly309Arg) are frequent mutations in the Netherlands, but are also found in other populations [ 20 , 21 , 22 , 23 ]. Chinese patients from Taiwan share a common c.1935C>A (p.Asp645Glu) mutation [ 24 ]. The fascinating story about the origin of the most common African-American mutation, c.2560C>T (p.Arg854Ter), brings us back to the population of north Central Africa and to the slave trade to the Americas [ 25 ].
Two sequence variants, c.1726G>A and c.2065G>A, are known to cause pseudodeficiency, a condition associated with low levels of GAA activity but not with clinical disease. The relatively high frequency of pseudodeficiency in Asian populations may increase false-positive results in newborn screening (see below) [ 26 , 27 , 28 ].
Clinical Manifestations, Diagnostic Tools, and Differential Diagnosis
Pompe disease affects people of all ages with varying degrees of severity. The continuum of phenotypes creates some ambiguity when it comes to classifying different subtypes. Two broad types are recognized based on the onset of symptoms and the presence or absence of cardiomyopathy. The most severe form, referred to as classic infantile onset Pompe disease (IOPD), is characterized by the age of onset at ≤ 12 months, rapidly progressive hypertrophic cardiomyopathy, left ventricular outflow obstruction, hypotonia and muscle weakness, respiratory distress, and progressive loss of independent ventilation. Breathing difficulties, feeding problems, and macroglossia are common manifestations. Motor development is significantly delayed, and major developmental milestones, such as the ability to roll over, sit, or stand, are often not achieved. Only a small percentage of untreated patients survive beyond 1 year of age; the main cause of death is cardiac and respiratory failure [ 29 , 30 ]. A subset of patients with similar clinical presentations during the first year of life but less severe cardiomyopathy (and absence of left ventricular outflow obstruction) is referred to as nonclassic IOPD [ 31 ]. If left untreated, severe muscle weakness leads to respiratory failure by early childhood. The term “atypical” IOPD is used by some clinicians to describe the patients who present within the first year of life without cardiomyopathy; however, the same terminology is often used to describe nonclassic IOPD. No doubt, this inconsistency will be resolved at some point.
Less devastating late-onset Pompe disease (LOPD) manifests any time after 12 months of age, usually without significant cardiac involvement. Late-onset patients commonly present with the symptoms of proximal limb-girdle myopathy. The progression of the symptoms is relatively slow but ultimately leads to profound muscle weakness and wasting, wheelchair dependency, and respiratory failure due to the involvement of the diaphragm. A history of “not being able to keep up with others” during physical activities may help clinical diagnosis in teenagers or adults. The introduction of enzyme replacement therapy (ERT) and a growing scientific interest brought more attention to the disease, and many additional symptoms came to light: dysarthria and dysphagia, osteoporosis, scoliosis, sleep apnea, small fiber neuropathy, hearing loss, impaired gastric function, urinary tract and anal sphincter involvement, and pain and fatigue, as well as a risk of cardiac arrhythmia and cerebral and intracranial aneurysms [ 32 ]. These findings emphasize the multisystem nature of Pompe disease.
Diagnostic Tools
Generally, the serum CK activity is elevated in Pompe patients, but a normal CK value in LOPD does not exclude the diagnosis. Other enzymes, such as aminotransferase (AST), alanine aminotransferase (ALT), and lactate dehydrogenase (LDH), are often elevated [ 29 , 33 ]. Most Pompe disease patients have elevated urinary glucose tetrasaccharide (Glc 4 ) levels which are higher in infants than in adults. This test can be useful for supporting the diagnosis and for monitoring the effects of ERT. Chest X-rays reveal massive cardiomegaly in IOPD, and cardiac evaluation includes an electrocardiogram (ECG) and echocardiography (Echo). ECG shows a short P-R interval, tall QRS complexes, and increased QT dispersions; Echo reveals increased left ventricular wall thickness and mass with or without left ventricular outflow tract obstruction [ 34 ].
The pulmonary function in late-onset cases is evaluated by measuring maximum inspiratory pressure (MIP), maximum expiratory pressure (MEP), forced vital capacity (FVC), and vital capacity (VC). The vital capacity is measured in the upright and supine positions; the latter helps evaluate the degree of diaphragmatic deficiency [ 34 , 35 ].
Magnetic resonance imaging (MRI) can be helpful for evaluating the extent and localization of muscle changes in patients with LOPD. Although the enzyme activity is deficient in all muscles, some muscle groups are relatively well preserved even during advanced stages of the disease. MRI can also help identify the site for a muscle biopsy.
By histology, muscle biopsies show vacuolar myopathy, the extent of which usually correlates with the severity of clinical symptoms. Vacuoles are diastase sensitive and positive for periodic acid-Schiff (PAS) and acid phosphatase, a combination which confirms the nature of the storage material and its lysosomal origin. The diagnostic value of muscle biopsies in adult patients is rather limited and rightly questioned because different muscle groups, and even fibers within the same muscle group, exhibit highly variable pathology [ 36 ]. However, since LOPD often presents the diagnostic challenge, muscle biopsy may be helpful. Of note, histological identification of acid phosphatase-positive lipofuscin inclusions was suggested as a new diagnostic marker, particularly in adult patients [ 37 , 38 ].
Most importantly, the diagnosis is established by demonstration of deficiency of GAA enzymatic activity. The activity can be measured in blood, dried blood spots, cultured skin fibroblasts, or in a muscle biopsy. In classic IOPD, the enzyme activity is absent or almost absent (< 1%), whereas low levels of residual activity, up to approximately 30% of normal, are usually measurable in all other clinical forms [ 39 , 40 ]. Nowadays, most academic and nonacademic institutions perform GAA mutation analysis not only to confirm the diagnosis but also to assess the genotype−phenotype correlation, to identify carriers within families, and to provide genetic counseling.
Differential Diagnosis
A number of rare diseases presenting with cardiomyopathy, hypotonia, and myopathy in infancy should be considered. These include Werdnig−Hoffman disease, Danon disease, glycogenoses types III and IV, nemaline myopathy, myofibrillar myopathy, and mitochondrial myopathies. Importantly, newborn screening eliminates the need for differential diagnosis in IOPD: clinical findings plus a decreased enzyme activity are sufficient to confirm the diagnosis.
The diseases that may resemble LOPD include limb-girdle muscle dystrophy, Duchenne muscular dystrophy and Becker muscular dystrophy, facioscapulohumeral muscular dystrophy (FSHD), scapuloperoneal syndromes, rigid spine syndrome, myasthenia gravis, polymyositis, fibromyalgia, chronic fatigue syndrome, and glycogenoses types V and VI [ 32 ].
Pathogenesis of Muscle Damage
The loss of muscle structure and muscle force have long been attributed to the progressive enlargement of glycogen-filled lysosomes in the intermyofibrillar space followed by lysosomal rupture, accumulation of cytoplasmic glycogen, and displacement of the myofibrils [ 41 , 42 ]. In retrospect, this view seems overly simplistic and inadequate because it does not take into consideration any of the secondary events that may occur as a result of accumulation of unmetabolized substrates in the lysosomes. Recently, it became abundantly clear that a number of pathogenic mechanisms, such as autophagy, calcium homeostasis, oxidative stress, and mitochondrial abnormalities, all contribute to tissue damage in Pompe disease as well as in other LSDs.
Defective autophagy has emerged as a critical common feature of many LSDs [ 43 ]. Autophagy (the term literally means “self-eating”) is a recycling system of lysosomal delivery and degradation of intracellular components. At least three autophagic pathways have been described based on the route by which the cargo enters the lysosome [ 44 ]. In microautophagy, a direct invagination of the lysosomal membrane brings the cargo into the lysosomal lumen [ 45 ]; in chaperone-mediated autophagy, molecular chaperones deliver a subset of cytosolic proteins to the lysosome through the lysosome-associated membrane protein type 2A (LAMP-2A) [ 46 ]; macroautophagy involves sequestration of various cytosolic constituents into newly formed double-membrane vesicles (autophagosomes) which fuse with and discharge their content into lysosomes for breakdown and recycling [ 47 ]. Macroautophagy (traditionally referred to as “autophagy”) is thought to be the predominant form of autophagy, and it is profoundly dysregulated in Pompe disease. Multiple methods and markers for studying autophagy are now available [ 48 ], but perhaps the most important is the protein, MAP1LC3, commonly referred to as LC3, which is a highly specific marker of autophagosomes [ 49 ].
The morphological evidence for abnormal autophagy in muscle biopsies from adult Pompe disease patients was first reported by Dr. Engel [ 50 ]. However, this pathology and its contribution to the pathogenesis of the disease have largely been ignored. Later on, in preclinical trials, poor skeletal muscle response to ERT was, at least in part, linked to the presence of large areas of autophagic accumulation (autophagic build-up) reminiscent of those described by Engel in adult Pompe patients [ 51 ]. The extent of this pathology became clear when single muscle fibers were immunostained for LAMP1 (a marker for lysosomes) and LC3; the core of the fibers contained large areas composed of numerous autophagosomes, clustered late endosomes and lysosomes with broken borders, and autofluorescent material, as well as other cellular debris of unknown origin. In addition, the area is filled with undigested autophagic substrates, such as p62/SQSTM1 and potentially toxic ubiquitinated protein aggregates [ 52 , 53 ]. The presence of large pools of autophagic debris in skeletal muscle and the impact of this pathology on therapy warrant the classification of Pompe disease into a group of disorders known as autophagic myopathies [ 54 ]. Furthermore, it has been shown that the autophagic build-up affects the trafficking and delivery of the recombinant enzyme to the lysosome [ 55 , 56 ]. Thus, in Pompe disease, a profoundly disordered intracellular recycling system appears to be an important contributor to muscle weakness and incomplete response to treatment.
Impaired autophagy is directly related to mitochondrial abnormalities since damaged mitochondria are removed through the autophagic pathway, a process known as mitophagy [ 57 ]. Indeed, mitochondrial alterations are observed in muscle biopsies in the majority of Pompe patients [ 42 , 58 ]. A profound dysregulation of Ca 2+ homeostasis and multiple mitochondrial defects, such as a decrease in mitochondrial membrane potential, mitochondrial Ca 2+ overload, an increase in reactive oxygen species, and an increase in caspase-independent apoptosis, were reported in GAA knockout (KO) mice and in primary muscle cells from Pompe disease patients [ 59 ]. Accumulation of excess Ca 2+ in electron-dense globular bodies within the area of autophagic build-up was also reported on computerized tomographic (CT) images of severely affected muscles in children with Pompe disease [ 60 ]. These electron-dense globular bodies, lipofuscin inclusions, are commonly found in diseased muscle [ 38 , 58 ]. Progressive deposition of lipofuscin in the lysosomes and autophagosomes further diminishes the degradative capacity of the lysosomes leading to a decrease in the autophagic turnover of damaged mitochondria, generation of reactive oxygen species, and formation of oxidized proteins and aggregates, thus perpetuating the “vicious circle.”
Thus, large clusters of noncontractile material, such as glycogen-laden lysosomes, lakes of cytoplasmic glycogen, autophagic debris, and lipofuscin, interrupt the contractile machinery leading to muscle damage and decline of muscle function [ 61 , 62 ]. Yet another layer in the pathogenic cascade is a dysregulation of the mTOR (mammalian target of rapamycin) signaling pathway in diseased cells. mTOR kinase is a potent anabolic regulator, and the lysosome serves as a platform for its activation [ 63 ]. Furthermore, this kinase exerts control over muscle mass [ 64 ]. The diminished mTOR activity and the failure to shuttle to and from the lysosomes in response to cellular stress were shown to contribute to muscle wasting in Pompe disease [ 65 ].
Enzyme Replacement Therapy
Ert in infants.
A breakthrough in treating LSDs came with the serendipitous discovery of the lysosomal enzyme secretion–reuptake pathway: Neufeld and colleagues demonstrated that cultured fibroblasts from patients with two different lysosomal storage disorders, Hunter and Hurler’s disease, were able to correct each other [ 66 , 67 ]. Subsequent studies showed that secreted lysosomal enzymes can enter the endocytic pathway and reach lysosomes via the cation-independent-M6P receptors (CI-MPR) on the cell surface [ 68 ]. This naturally occurring metabolic cross-correction suggested that LSDs may be amenable to therapy with exogenously administered functional enzymes, a concept which became known as enzyme replacement therapy.
The success of ERT for the non-neuropathic form of Gaucher disease made ERT an obvious approach to explore for other LSDs. Pompe disease, however, is the only lysosomal storage disorder in which muscle is the primary target. The first ERT clinical trial in infants was conducted by the Rotterdam group using recombinant human acid alpha-glucosidase (rhGAA) from transgenic rabbit milk [ 69 , 70 ] followed by the Duke group using the enzyme produced and purified from CHO (Chinese hamster ovary) cells [ 71 ]. The production of the milk product was later discontinued, and all surviving patients were transitioned to CHO cell-derived enzyme. Several other trials including two pivotal company-sponsored multicenter, multinational, open-label studies of rhGAA safety and efficacy in infants younger than 6 months (18 pts; AGLU 1602) and infants and children between the ages 3 to 43 months with cardiac involvement and onset of symptoms during infancy (21 pts; AGLU 1702) led to the approval of the first specific treatment for Pompe disease. In 2006, human recombinant acid alpha-glucosidase (alglucosidase alfa; marketed as Lumizyme within the USA and as Myozyme outside of the USA; Sanofi Genzyme, Cambridge, MA) received broad-label marketing approval in Europe and in the USA. ERT is currently the standard of care to treat Pompe disease, and it is the first instance of using recombinant enzyme to treat skeletal muscle.
The results of the clinical trial of 18 nonventilator-dependent infants (< 6 months of age) treated for 52 weeks clearly demonstrated that the therapy markedly improved cardiomyopathy and cardiac function, dramatically reduced the risk of death (by 99%) and the risk of invasive ventilation (by 92%) compared to the outcomes in an untreated historic cohort. However, significant gross motor milestones were achieved only in a subgroup of patients [ 72 ]. During a longer follow-up period of up to 3 years, the survival rate dropped to 67.5%, the number of patients who became ventilator dependent rose to 50%, and 40% of those who initially learned to walk continued to do so [ 73 ]. Similarly, the second trial of 21 patients showed that the drug significantly improved cardiac function, reduced the risk of death (by 79%), and reduced the need for invasive ventilation (by 58%) [ 74 ].
The outcome of 20 infantile patients treated in the UK from 2000 to 2009 was poorer than in the pivotal clinical trials: 35% died at a median age of 10 months and 30% were ventilator dependent [ 75 ]. Another retrospective analysis of 23 patients with classic infantile form treated for a period of at least 30 months in Germany between 2003 and 2010, again, yielded more sobering results: 60% of the patients died or became ventilator dependent; 30.5% made no motor progress and approximately half of the patients with a positive initial response deteriorated during the course of the disease [ 76 ].
Apart from the variability in the results of clinical trials, the studies outside the clinical trials, and multiple case reports on the effect of ERT in patients with classic infantile form, a number of common findings can be drawn. No question, the therapy has changed the natural course of the disease and has significantly extended the lifespan of infants; all patients had striking and sustained improvement in cardiac parameters with marked decreases in left ventricular mass index and left ventricular wall thickness, correction of abnormal ECG parameters, and improvement of cardiac function; many patients achieve major milestones of motor development. It is, however, equally clear that most long-term survivors still carry the burden of the disease. The emerging new phenotype includes gross motor weakness, hearing loss, ptosis, facial muscle weakness, speech difficulties, dysphagia that can lead to aspiration risk, arrhythmias, recurrent pneumonias, osteopenia, and orthopedic deformities [ 76 , 77 , 78 ].
These data suggest that infantile Pompe disease still remains a life-threatening condition. Many patients do not survive ventilator free beyond 3 years of age, and respiratory infections and invasive ventilation can be life threatening. A recent study reported improved outcomes in four patients receiving higher and more frequent dosing of the drug (40 mg/kg/week) instead of the currently recommended 20 mg/kg/every other week [ 79 ]. Nevertheless, even the most optimally treated infants tend to develop motor problems [ 28 ]. On top of that, brain magnetic resonance imaging (MRI) and neuropsychological tests revealed cerebral white matter abnormalities and different degrees of cognitive decline in long-term survivors; these are the results of a new prospective study of a group of ERT-treated patients with classic IOPD [ 80 ]. These data underscore yet another limitation of ERT—the inability of the recombinant enzyme to cross blood-brain barrier.
Another common thread that emerges from multiple studies is that the therapy is negatively affected by immune responses. Nearly all Pompe patients develop antibodies to the exogenous protein, but the impact of the immune response is particularly detrimental in classic infantile patients who do not produce any endogenous acid alpha-glucosidase. These patients, referred to as cross-reactive immunologic material negative (CRIM-negative), develop high antibody titers associated with clinical decline often leading to death despite ongoing therapy [ 81 , 82 ]. In a retrospective study of the influence of CRIM status on outcomes in patients receiving the drug, all 21 CRIM-negative patients were deceased or invasively ventilated by age 27.1 months [ 83 ]. Several protocols have been introduced for tolerance induction in CRIM-negative patients [ 84 , 85 ]. The most common is a combination of rituximab with methotrexate with or without intravenous gamma globulins. The addition of bortezomib (Velcade) to immunomodulatory regimens was shown to be an effective and safe treatment strategy in a group of infantile patients with an established immune response associated with clinical decline [ 86 ]. High antibody titers were also reported in CRIM-positive adult patients [ 87 , 88 ].
The consensus is that the timing of ERT initiation is of critical importance for the outcome of therapy—the earlier the better. The start of therapy in IOPD before 6 months of age has long met the definition of “early.” However, this paradigm was changed with the introduction of newborn screening program, and the current view is that the best time frame for the initiation of treatment is within the first days after birth [ 89 ]. Newborn screening (NBS), which marks a new era in Pompe field, will be discussed in more detail (see below).
ERT in Children and Adults
The only randomized, double-blind, placebo-controlled phase III clinical trial of alglucosidase alfa for the treatment of LOPD was performed in children and adults (over 8 years of age). This multicenter, multinational study involved 90 patients, 60 of whom received the drug and 30 received placebo over the course of 78 weeks (late-onset treatment study/LOTS) [ 90 ]. All patients were ambulatory and free of invasive ventilation. Inclusion criteria were the ability to walk at least 40 m and FVC in upright position between 30 and 80% of normal. This trial also included a prior observational study [ 91 ] and an extension study [ 92 ] . The alglucosidase alfa-treated patients showed improvements in walking distance [a mean increase of 28.1 m on the 6-minute walk test (6MWT)] and stabilization of respiratory function. The trial also revealed a great deal of variability in the response to treatment, and some patients continued to deteriorate despite therapy.
Since the introduction of ERT, a number of observational open-label studies and individual or small series case reports on the effect of therapy in LOPD have been published [ 93 , 94 , 95 , 96 ]. These studies provided evidence of a beneficial effect of ERT at a group level, but the response to therapy varied significantly among patients. In most patients, the treatment was associated with improved ambulation, some degree of modest motor function improvement, an increase in distance walked on the 6MWT, and a modest increase or stabilization of pulmonary function measured by FVC. During the follow-up period, many adult patients maintained a plateau after the initial improvement. The results of these studies are summarized in a review by Toscano and colleagues [ 97 ], which covers 21 studies and provides an overview of clinical data from 368 Pompe patients (2 years of age or older) treated for a period of time from less than a year to over 3 years.
A more recent literature review [ 98 ] added new reports including the large UK study of 62 patients [ 99 ] and accounted for patients overlap between the studies. The authors used a variety of analytic methods to adjust for repeat measures and different follow-up time. The updated review covers 438 LOPD patients, who were monitored for 3 to 48 months. The major conclusions of this study are as follows: the mortality rate is fivefold lower in treated compared to untreated patients (hazard ratio, 0.21; 95% credible interval (CrI): 0.11, 0.41]; at the group level, in treated patients, FVC improved rapidly within the first few months of treatment (an average increase of 1.4%) and then gradually returned to baseline, followed by a slight decline after 2 to 3 years, whereas in untreated patients, a continuous decline is observed. The improvement in 6MWT was most pronounced over the first 20 months of treatment and was sustained over time. ERT was also shown to reduce fatigue [ 100 ]. The positive effect of ERT on survival was first demonstrated in a prospective international observational study of 283 adult patients [hazard ratio, 0.41; 95% confidence interval (CI), 0.19 to 0.87] [ 101 ]; an estimated hazard ratio, as mentioned above, was even lower when the survival results were estimated across all selected studies [ 98 ].
Thus, the development of enzyme replacement therapy was unquestionably a major scientific and commercial achievement in the history of Pompe disease. The introduction of ERT dramatically changed the natural course of the disease in infants and resulted in much longer survival. The most reliable effect of ERT has been on cardiac pathology and function regardless of disease severity. In contrast, the skeletal muscle response is variable and less impressive despite a high dose of the drug compared to those in other lysosomal storage diseases.
Experimental Therapies Designed to Enhance the Effect of ERT
Effective ERT depends on the M6P content of the recombinant enzyme and on the abundance of CI-MPR on the target tissue. M6P groups are critical for efficient uptake and lysosomal delivery of the recombinant enzyme. The limited effect of ERT in Pompe skeletal muscle has been mainly attributed to both low number of M6P groups on the rhGAA and the low expression of the receptor on the cell surface of muscle cells [ 102 ]. Several approaches designed to improve traditional ERT are currently under investigation.
One of these is aimed at enhancing the enzyme delivery by increasing the number of M6P residues on the recombinant enzyme. Neo-GAA (Sanofi Genzyme) is a second-generation alglucosidase alfa that has an increased affinity for the CI-MPR, and is in a phase 3 randomized, multicenter, multinational, double-blinded study ( NCT02782741 ). In preclinical studies, this modified enzyme showed greater efficacy compared to alglucosidase alfa and reduced glycogen to similar levels at a much lower dose [ 103 ]. Another experimental drug, ATB200, is a novel rhGAA with a high content of M6P- and bis-M6P glycan (Amicus Therapeutics, Inc.). The effect of ATB200 was tested in KO mice, and it caused much improved glycogen clearance compared to the current drug (our unpublished data).
Since the CI-MPR also binds insulin-like growth factor II (IGFII) [ 104 ], glycosylation-independent lysosomal targeting (GILT) technology has been developed for the improved uptake of IGFII-tagged proteins via CI-MPR without the need for M6P residues [ 105 ]. A novel fusion protein between rhGAA and IGFII has been produced and successfully tested in Pompe mice [ 106 ]; however, the drug was withdrawn from phase 3 clinical trials due to safety concerns ( NCT01924845 ).
An attractive experimental approach involves the grafting of a synthetic analogue of M6P onto rhGAA leading to a significant increase in the affinity of the recombinant enzyme to the M6PR without changes in the catalytic activity. This glyco-engineered enzyme greatly improved muscle pathology and function even in hard-to-treat old KO mice, whereas rhGAA was inactive [ 107 ]. Another glyco-engineered rhGAA with a high content of M6P glycan has been recently successfully tested in fibroblasts from Pompe patients [ 108 ].
Upregulation of CI-MPR receptor by the β2-agonist clenbuterol or albuterol was shown to increase efficacy of ERT in a mouse model [ 109 , 110 ]. A pilot open-label study of adjunctive albuterol therapy in LOPD patients with no improvements on ERT (following initial stabilization) revealed safety and potential efficacy of this strategy [ 111 ].
An emerging strategy for the treatment of Pompe disease as well as other LSDs is chaperone therapy which relies on the ability of small molecule pharmacological chaperones to promote folding, stability, and lysosomal trafficking of chaperone-responsive mutant enzymes. In addition, the chaperones have been shown to have a stabilizing effect on the recombinant enzymes leading to their improved pharmacokinetics and pharmacodynamics [ 112 ]. Indeed, improved stability of alglucosidase alfa in blood was observed in Pompe disease patients receiving ERT in combination with iminosugar N -butyldeoxynojirimycin [ 113 ]. A similar approach has been used by combining the iminosugar miglustat (also known as AT2221) with ATB200. The effect of coadministered AT2221 on ATB200 is being investigated in a phase 2 clinical trial in LOPD (NCT02675465).
Experimental Therapies in Preclinical Studies
Substrate reduction therapy (SRT) , by definition, offers a new approach designed to diminish or even prevent accumulation of glycogen. Inhibition of glycogenin or glycogen synthase, the two major enzymes involved in glycogen synthesis, reduced lysosomal glycogen accumulation and lysosomal size in GAA-deficient myoblasts. Inhibition of glycogen synthase in vivo in KO mice reversed cardiac abnormalities, reduced glycogen storage and autophagic build-up, and improved exercise capacity [ 114 , 115 ].
The genetic suppression of autophagy in KO mice by selective inactivation of a critical autophagic gene in skeletal muscles resulted in a significant decrease in the amount of stored lysosomal glycogen, supporting the idea that the autophagic pathway is, at least partially, responsible for the delivery of glycogen to the lysosomes. Once the autophagic build-up was eliminated, ERT worked remarkably well as was shown by a nearly complete clearance of lysosomal glycogen [ 116 ].
Stimulation of lysosomal exocytosis is an exciting recently proposed approach to therapy for LSDs. This approach takes advantage of the intrinsic ability of lysosomes to undergo exocytosis, a calcium-dependent process of lysosomal docking to the plasma membrane, followed by fusion with the membrane and discharge of the lysosomal content outside the cell [ 117 , 118 ]. Inducing lysosomal exocytosis became possible with the discovery of the function of the transcription factors, TFEB and TFE3, in regulating lysosomal and autophagosomal biogenesis [ 119 , 120 , 121 ]. Overexpression of TFEB or TFE3 in Pompe muscle cells induced lysosomal exocytosis and promoted glycogen clearance in KO mice, thus circumventing the major obstacle of the current therapy—inefficient enzyme delivery to skeletal muscle [ 55 , 121 ]. However, systemic AAV-mediated delivery of TFEB to skeletal muscle of KO mice yielded somewhat disappointing results, although an improvement of muscle pathology and function was observed [ 122 ].
Gene Therapy Strategies
A potential alternative to ERT is gene therapy. Gene therapy is the delivery of a functional copy of a gene, deemed the transgene, into a patient’s tissue without replacing or removing the mutated copy of the gene harbored within the patient’s own genome. Gene therapy is currently being developed for treatment of Pompe disease, as well as other genetic disorders, and relies on delivery of the transgene within a viral vector.
Initial studies using adeno- (Ad), adeno-associated viruses (AAV), and retroviruses demonstrated the feasibility of gene therapy for Pompe disease [ 123 , 124 , 125 , 126 ]. Systemic correction of muscle pathology in KO mice was achieved by hepatic targeting of a modified Ad-virus encoding human GAA [ 125 ]. This study was the first to demonstrate that liver can be a source of secreted GAA for cross-correction of skeletal muscle. Retroviral vectors, such as lentiviruses, have been successfully used in vitro in GAA-deficient cell lines and in vivo in KO mice [ 127 , 128 , 129 ]. However, concerns about the safety of retroviral vectors in clinical studies remain, as they can integrate into the genome at random sites and cause unintended mutations in or knockout of bystander genes. Additionally, long terminal repeats (LTR) at the ends of the viral genome can promote expression of nearby oncogenes present in the patient’s own genome. AAV vectors are now preferred because they are nonpathogenic, can infect both replicating and nonreplicating cells, require a helper virus for infection, have low immunogenicity when compared to other vectors, and are available in multiple serotypes, with each serotype having a specific tissue tropism, allowing for more specific targeting of the desired tissue [ 130 ]. Although wild-type AAVs integrate into the genome, engineered recombinant AAVs (rAAVs) that lack the rep protein do not, thus mitigating some clinical safety risks. Thus, AAV vectors have become the accepted delivery mechanism for Pompe disease gene therapies under investigation in KO mice and in clinical trials.
One strategy for Pompe disease patients is to target muscles with direct injections of rAAV expressing GAA protein. This approach resulted in increased expression of GAA protein in KO mice [ 126 , 131 ], but glycogen reduction was restricted to the injected muscle, without significant improvement in other muscles. Furthermore, recent studies have demonstrated that targeting muscle alone may not be enough to fully restore muscle function. Preclinical studies and autopsy reports on ERT-treated children confirmed the accumulation of glycogen in motoneurons [ 132 , 133 , 134 ]. Neurological deficits caused by the excess glycogen accumulation in the central nervous system (CNS) and peripheral nervous system contribute to muscle dysfunction. In a series of studies using spinal, intrathecal, or intracerebroventricular delivery of AAV-GAA, neuromuscular improvement was observed, although muscle glycogen storage was not affected by the treatments [ 135 , 136 , 137 ]. Better outcomes were achieved by systemic delivery of AAV vectors of different serotypes. AAV serotypes have been identified in dozens of preclinical studies that improve efficacy and reduce immunogenicity. These studies demonstrated advantages of gene therapy compared to ERT, but the immune response remained an obstacle, particularly because high vector doses are required to achieve therapeutic efficiency.
In general, immune reactivity to the viral capsid and the transgene product has remained a major challenge to translating advances in AAV-mediated gene therapy to the clinic. Immune response may occur if a patient has previously been infected with AAV of the same serotype earlier in life or as a cause of previous ERT. Patients with pre-existing immunity to the wild-type AAV virus are less likely to benefit from AAV vector-based therapies. An immune response against the AAV-encoded transgene products may develop in the course of gene therapy. However, it is important to note that such reactions have been controlled during clinical trial using B-cell depletion by the drug rituximab to reduce reactivity to both the AAV capsid and to the GAA transgene [ 138 ]. The numerous studies on immune reactions during Pompe gene therapy have been reviewed elsewhere [ 139 , 140 , 141 ]. Here, we will focus on preclinical studies that have led to clinical trials.
Systemic and intradiaphragmal delivery of rAAV1-hGAA was shown to improve respiratory function in KO mice [ 142 , 143 ]. Subsequent studies demonstrated the capacity of AAV for retrograde movement and transduction of phrenic motoneurons: intralingual delivery of AAV produced temporary correction of motoneuron pathology in KO mice [ 144 ]. Based on these preclinical studies, the first-in-human trial of diaphragmatic gene therapy (AAV1-CMV- GAA ) was conducted in five children with IOPD who required assisted ventilation prior to the study. This trial has been recently completed [ 145 , 146 , 147 , 148 ]. The study demonstrated the safety of the AAV treatment, but the clinical outcome was minimal: no improvements in muscle function or dissemination of the GAA transgene were detected outside of the injected tissue. However, patients did exhibit an increase in tidal volume and the period of time that they could tolerate unassisted breathing. Additional clinical trial is planned ( ClinicalTrials.gov ID: NCT02240407 ). A recombinant AAV vector carrying the codon-optimized acid alpha-glucosidase gene under control of a human desmin promoter will be used (rAAV2/9-DES-hGAA). Because desmin is highly expressed in muscle, this improves expression levels of the GAA transgene when compared to other AAV vectors for Pompe disease. Neural and cardiorespiratory function improved following systemic or intrapleural delivery of this vector in KO mice [ 149 , 150 ]. The proposed clinical trial will evaluate the toxicology, biodistribution, and potential for readministration of rAAV9-DES-hGAA injected intramuscularly into the tibialis anterior muscle using an immune modulation strategy [ 151 ].
Another approach is liver-targeted gene therapy. To enhance systemic delivery and expression of GAA protein, investigators have harnessed the high metabolic capacity of the liver to produce and secrete the GAA protein. This strategy relies on infection with AAV8, which has a tropism for hepatic cells. Researchers at Duke University developed such a strategy to enhance and potentially replace ERT. Systemic injection of a modified AAV8 vector containing liver-specific promoter (AAV2/8-LSPhGAA) induced immune tolerance to rhGAA and improved the efficacy of ERT in KO mice when administered at a low dosage of viral particles [ 152 ]. The appeal of this approach, termed “immunomodulatory gene therapy” [ 152 ], is twofold: it induces immune tolerance to GAA by activating regulatory T cells and can provide a stable expression of GAA in liver, thus converting liver into a depot for continuous secretion of GAA and cross-correction in distant organs. Preclinical studies have demonstrated that the secreted GAA is taken up by cardiac and skeletal muscles leading to glycogen reduction and improved muscle function [ 141 , 153 ]. A clinical trial which is scheduled to begin in the fall 2018 will explore the safety of liver-targeted gene therapy.
A more recent preclinical study sought to optimize the liver-directed strategy by testing genetically engineered GAA transgenes (that were codon optimized and contained small deletions within the progene and modified secretion signals) for their ability to be expressed by hepatocytes and produce the highly secretable GAA protein [ 154 ]. The authors showed in further in vivo experiments that these transgenes, delivered by liver-tropic rAAV, resulted in high levels of secreted GAA, low immunogenicity, and metabolic cross-correction in muscle, central nervous system, and spinal cord. Experiments in nonhuman primates demonstrated the safety of this approach and confirmed that the liver-secreted GAA is efficiently taken up by peripheral tissues [ 154 ].
In comparison to ERT, gene therapy could offer several benefits to Pompe patients. Gene therapy could be more convenient and cheaper because it would potentially require as few as one treatment for the entire lifespan of the patient. In contrast, the currently available ERT must be delivered biweekly via IV drip over the course of 6 to 7 h per treatment, causing discomfort and inconvenience to the patient. Importantly, gene therapy has the potential to be more effective than ERT, particularly if administered early during disease development. This increased efficacy includes the ability of certain AAV serotypes to cross the blood-brain barrier as has been demonstrated in the correction of neurological symptoms associated with mucopolysaccharidosis IIIB in mice [ 155 ].
While gene therapy for Pompe disease is promising, it is likely that a cure will eventually arrive with genome editing via the CRISPR/Cas system. Current versions of this system rely on delivery of the Cas9 protein and an RNA guide sequence to target and edit mutations in the genome. The gene may be edited by either nonhomologous end joining (NHEJ) or homology-directed repair (HDR) [ 156 ]. NHEJ leads to random mutations in the targeted portion of the gene, with the intention that a stop codon will arise causing the protein to no longer be expressed. NHEJ may be similarly applied at splice sites to edit out mutated exons for treatment of other diseases that do not require expression of the full-length protein for functionality. Modification of a splice site using NHEJ has been done successfully to correct disease pathology in mdx mice, the animal model for Duchenne’s muscular dystrophy [ 157 , 158 , 159 , 160 ] and in dy 2J /dy 2J mice, the animal model for congenital muscular dystrophy type 1A [ 161 ].
However, CRISPR strategies using NHEJ would not correct the site-specific mutations found in the majority of Pompe cases, in which restoring a functional full-length GAA protein would be desired. Instead, site-specific corrections via HDR or other methods, such as base editors, would be necessary. HDR-mediated CRISPR strategies are currently somewhat inefficient in muscle cells because DNA repair proteins that are required for HDR strategies are not highly expressed [ 162 ]. However, advancements in the targeting and efficiency of genome editing in muscle would facilitate a cure for many other muscle-wasting diseases, representing a focused strategy for the potential cure of many diseases with a single approach.
Newborn Screening
The first nationwide newborn screening program for Pompe disease was established in Taiwan over a decade ago [ 163 ]. The screening (conducted between 2005 and 2007) covered close to half of all the newborns in the country and measured GAA activity in dried blood spots (DBS). The number of diagnosed IOPD cases was similar to the number of infants diagnosed clinically among the unscreened control population. Although the classic infantile form of Pompe disease in theory does not present major diagnostic challenge, delays in clinical diagnosis are unavoidable. Indeed, NBS resulted in earlier diagnosis (less than 1 month of age) compared to 3 to 6 months in the control group. The studies that followed clearly demonstrated the long-term benefits of early diagnosis and early initiation of ERT in classic infantile disease [ 28 , 164 , 165 ]. Any delay negatively affects the treatment outcome and even a few days can make a difference as shown in a group of patients identified through NBS conducted in Taiwan between 2008 and 2012 [ 166 ]. High frequency of pseudodeficiency in the Taiwanese population (p.G576S; 14.5%) [ 27 ] complicates the screen and could increase false-positive results. Therefore, to promote early treatment for IOPD, Yang et al. developed a diagnostic protocol which included a combination of low GAA activity in DBS (≤ 0.5 μmol/L/h) with hypotonia, elevated CK (≥ 250 U/L), and high left ventricular mass index (LVMI ≥ 80 g/m 2 ). The authors also argue that the benefits of early ERT for patients with highly suspected IOPD outweigh the low risk of adverse effects associated with the administration of the drug [ 166 ]. Thus, the importance of NBS for early diagnosis and treatment of IOPD is unquestionable.
However, screening will identify newborns with all forms of the disease, and most cases will be LOPD since this form is more prevalent [ 167 , 168 ]. These cases require decisions regarding the frequency of monitoring, the methods of follow-up assessments, and the timing of initiation of life-long treatment of individuals with unpredictable age of onset, not to mention psychological harm associated with the diagnosis and uncertainty. Because of these challenges, it took a while to convince policy makers to add Pompe disease to newborn screening panels. Again, Taiwanese experience showed that the screening and the subsequent follow-up of patients with LOPD allowed identification of the earliest manifestations of the disease and an early start of therapy leading to better outcomes [ 169 , 170 ].
It is important to remember that because of the range of clinical presentations and a variable age at onset of the symptoms, patients with LOPD can remain undiagnosed for years [ 171 ]. For those who have been through a diagnostic “odyssey,” the treatment may come too late. A recent prospective study by Rairikar et al. underscores the point: the authors followed up and evaluated the phenotype of infants identified through NBS, whose genetic makeup predicted late-onset disease based on the presence of a common “mild” leaky splice site mutation in the gene (c.-32-13T>G). When properly evaluated, compound heterozygotes had elevated CK and other biochemical parameters and exhibited symptoms, such as swallowing difficulties, limb-girdle weakness, and delayed motor milestones as neonates. Even when the mutation was present in homozygosity, infants had subtle signs of Pompe disease [ 172 ].
The recommendation by the Advisory Committee on Heritable Disorders in Newborns and Children (ACHDNC) to add Pompe disease to RUSP (Recommended Uniform Screening Panel) was finally approved in March 2015 and implemented in several states in the USA: NY state, Illinois, Kentucky, Pennsylvania, Missouri, Ohio, Tennessee, and Washington state (pilot). Enzyme activity is measured in DBS using a fluorometric method [ 173 ], tandem mass spectrometry, or microfluidics combined with fluorometry [ 174 ]. The Pompe Disease Newborn Screening Working Group, an international group of experts in both NBS and Pompe disease, developed recommendations for confirmatory testing after positive NBS result and guidelines regarding monitoring and management of patients before and during ERT [ 40 , 89 ]. One of the unexpected findings from these studies is a much higher prevalence of the disease than previously recognized. The estimate is 1:27,800 (University of Washington), 1:8657 (Missouri), and 1:15,133 (Illinois) for all forms of the disease.
The development and introduction of enzyme replacement therapy for Pompe disease have changed the natural history of the disease, significantly extended the lifespan of patients, and improved morbidity. However, the results have not fully met expectations, and many patients continue to be burdened by the disease. The limitations of therapy have led to re-examination of the pathogenesis of muscle damage, stimulated efforts to enhance the efficacy of the current therapy, and to develop new approaches including gene therapy. The advent of newborn screening will allow for early diagnosis and initiation of therapy before irreversible changes have occurred. Finally, newborn screening revealed that this rare genetic disorder is not so rare after all.
Pompe JC. Over idiopatische hypertrophie van het hart. Ned Tijdschr Geneeskd 1932;76:304.
Google Scholar
Bischoff G. Zum klinischen Bild der Glykogen-Speicherungs-Krankheit (Glykogenose). Zeitschrift fur Kinderheikunde 1932;52:722.
Article Google Scholar
Putschar and Walter. Uber angeborene Glykogenspeicher-Krankheit des Herzens. “Thesaurismosis glycogenica” (v. Gierke). Beitr Pathol Anat Allg Pathol. 1932;90:222.
Cori GT. [Enzymes and glycogen structure in glycogenosis]. Osterreichische Zeitschrift fur Kinderheilkunde und Kinderfursorge 1954;10(1–2):38–42.
CAS PubMed Google Scholar
De Duve C, Pressman BC, Gianetto R, Wattiaux R, Appelmans F. Tissue fractionation studies. 6. Intracellular distribution patterns of enzymes in rat-liver tissue. Biochem J 1955;60(4):604–617.
Article PubMed Central Google Scholar
Hers HG. Alpha-glucosidase deficiency in generalize glycogen storage disease (Pompe's disease). Biochem J 1963;86:11.
Article CAS PubMed PubMed Central Google Scholar
Wisselaar HA, Kroos MA, Hermans MM, van Beeumen J, Reuser AJ. Structural and functional changes of lysosomal acid alpha-glucosidase during intracellular transport and maturation. J Biol Chem 1993;268(3):2223–2231.
Hoefsloot LH, Hoogeveen-Westerveld M, Kroos MA, van Beeumen J, Reuser AJ, Oostra BA. Primary structure and processing of lysosomal alpha-glucosidase; homology with the intestinal sucrase-isomaltase complex. EMBO J 1988;7(6):1697–1704.
Hermans MM, Wisselaar HA, Kroos MA, Oostra BA, Reuser AJ. Human lysosomal alpha-glucosidase: functional characterization of the glycosylation sites. Biochem J 1993;289(Pt 3):681–686.
Moreland RJ, Jin X, Zhang XK, et al. Lysosomal acid alpha-glucosidase consists of four different peptides processed from a single chain precursor. J Bio lChem 2005;280(8):6780–6791.
Article CAS Google Scholar
Kroos M, Hoogeveen-Westerveld M, van der Ploeg A, Reuser AJ. The genotype-phenotype correlation in Pompe disease. Am J Med Genet C: Semin Med Genet 2012;160(1):59–68. https://doi.org/10.1002/ajmg.c.31318 .
de Filippi P, Ravaglia S, Bembi B, et al. The angiotensin-converting enzyme insertion/deletion polymorphism modifies the clinical outcome in patients with Pompe disease. Genet Med 2010;12(4):206–211. https://doi.org/10.1097/GIM.0b013e3181d2900e .
Article CAS PubMed Google Scholar
Hoefsloot LH, Hoogeveen-Westerveld M, Reuser AJ, Oostra BA. Characterization of the human lysosomal alpha-glucosidase gene. Biochem J 1990;272(2):493–497.
Martiniuk F, Mehler M, Tzall S, Meredith G, Hirschhorn R. Sequence of the cDNA and 5′-flanking region for human acid alpha-glucosidase, detection of an intron in the 5′ untranslated leader sequence, definition of 18-bp polymorphisms, and differences with previous cDNA and amino acid sequences. DNA Cell Biol 1990;9(2):85–94.
Kuo WL, Hirschhorn R, Huie ML, Hirschhorn K. Localization and ordering of acid alpha-glucosidase (GAA) and thymidine kinase (TK1) by fluorescence in situ hybridization. Hum Genet 1996;97(3):404–406.
Huie ML, Chen AS, Tsujino S, et al. Aberrant splicing in adult onset glycogen storage disease type II (GSDII): molecular identification of an IVS1 (-13T-->G) mutation in a majority of patients and a novel IVS10 (+1GT-->CT) mutation. Hum Mol Genet 1994;3(12):2231–2236.
Boerkoel CF, Exelbert R, Nicastri C, et al. Leaky splicing mutation in the acid maltase gene is associated with delayed onset of glycogenosis type II. Am J Hum Genet 1995;56(4):887–897.
CAS PubMed PubMed Central Google Scholar
Raben N, Nichols RC, Martiniuk F, Plotz PH. A model of mRNA splicing in adult lysosomal storage disease (glycogenosis type II). Hum Mol Genet 1996;5(7):995–1000.
Musumeci O, Thieme A, Claeys KG, et al. Homozygosity for the common GAA gene splice site mutation c.-32-13T>G in Pompe disease is associated with the classical adult phenotypical spectrum. Neuromuscul Disord 2015;25(9):719–724. https://doi.org/10.1016/j.nmd.2015.07.002 .
Article PubMed Google Scholar
Hermans MM, De Graaff E, Kroos MA, et al. The effect of a single base pair deletion (delta T525) and a C1634T missense mutation (pro545leu) on the expression of lysosomal alpha-glucosidase in patients with glycogen storage disease type II. Hum Mol Genet 1994;3(12):2213–2218.
Hirschhorn R, Huie ML. Frequency of mutations for glycogen storage disease type II in different populations: the delta525T and deltaexon 18 mutations are not generally “common” in white populations. J Med Genet 1999;36(1):85–86.
Dagnino F, Stroppiano M, Regis S, Bonuccelli G, Filocamo M. Evidence for a founder effect in Sicilian patients with glycogen storage disease type II. Hum Hered 2000;50(6):331–333.
Herzog A, Hartung R, Reuser AJ, et al. A cross-sectional single-centre study on the spectrum of Pompe disease, German patients: molecular analysis of the GAA gene, manifestation and genotype-phenotype correlations. Orphanet J Rare Dis 2012;7:35. https://doi.org/10.1186/1750-1172-7-35 .
Article PubMed PubMed Central Google Scholar
Shieh JJ, Lin CY. Frequent mutation in Chinese patients with infantile type of GSD II in Taiwan: evidence for a founder effect. Hum Mutat 1998;11(4):306–312.
Becker JA, Vlach J, Raben N, et al. The African origin of the common mutation in African American patients with glycogen-storage disease type II. Am J Hum Genet 1998;62(4):991–994.
Kumamoto S, Katafuchi T, Nakamura K, et al. High frequency of acid alpha-glucosidase pseudodeficiency complicates newborn screening for glycogen storage disease type II in the Japanese population. Mol Genet Metab 2009;97(3):190–195. https://doi.org/10.1016/j.ymgme.2009.03.004 .
Labrousse P, Chien YH, Pomponio RJ, et al. Genetic heterozygosity and pseudodeficiency in the Pompe disease newborn screening pilot program. Mol Genet Metab 2010;99(4):379–383. https://doi.org/10.1016/j.ymgme.2009.12.014 .
Chien YH, Lee NC, Chen CA, et al. Long-term prognosis of patients with infantile-onset Pompe disease diagnosed by newborn screening and treated since birth. J Pediatr. 2015;166(4):985–991 e1–2. : https://doi.org/10.1016/j.jpeds.2014.10.068 .
van den Hout HM, Hop W, van Diggelen OP, et al. The natural course of infantile Pompe's disease: 20 original cases compared with 133 cases from the literature. Pediatrics 2003;112(2):332–340.
Kishnani PS, Hwu WL, Mandel H, Nicolino M, Yong F, Corzo D. A retrospective, multinational, multicenter study on the natural history of infantile-onset Pompe disease. J Pediatr 2006;148(5):671–676.
Slonim AE, Bulone L, Ritz S, Goldberg T, Chen A, Martiniuk F. Identification of two subtypes of infantile acid maltase deficiency. J Pediatr 2000;137(2):283–285.
Chan J, Desai AK, Kazi ZB, et al. The emerging phenotype of late-onset Pompe disease: A systematic literature review. Mol Genet Metab 2017;120(3):163–172. https://doi.org/10.1016/j.ymgme.2016.12.004 .
Winkel LP, Hagemans ML, Van Doorn PA, et al. The natural course of non-classic Pompe's disease; a review of 225 published cases. J Neurol 2005;252(8):875–884.
Kishnani PS, Steiner RD, Bali D, et al. Pompe disease diagnosis and management guideline. Genet Med 2006;8(5):267–288.
Johnson EM, Roberts M, Mozaffar T, Young P, Quartel A, Berger KI. Pulmonary function tests (maximum inspiratory pressure, maximum expiratory pressure, vital capacity, forced vital capacity) predict ventilator use in late-onset Pompe disease. Neuromuscul Disord 2016;26(2):136–145. https://doi.org/10.1016/j.nmd.2015.11.009 .
Vissing J, Lukacs Z, Straub V. Diagnosis of Pompe disease: muscle biopsy vs blood-based assays. JAMA Neurol 2013:1–5. https://doi.org/10.1001/2013.jamaneurol.486 .
Tsuburaya RS, Monma K, Oya Y, et al. Acid phosphatase-positive globular inclusions is a good diagnostic marker for two patients with adult-onset Pompe disease lacking disease specific pathology. Neuromuscul Disord 2012;22(5):389–393. https://doi.org/10.1016/j.nmd.2011.11.003 .
Feeney EJ, Austin S, Chien YH, et al. The value of muscle biopsies in Pompe disease: identifying lipofuscin inclusions in juvenile- and adult-onset patients. Acta Neuropathologica Communications 2014;2(1):2–17. https://doi.org/10.1186/2051-5960-2-2 .
Van der Ploeg AT, Reuser AJ. Pompe’s disease. Lancet 2008;372(9646):1342–1353.
Article PubMed CAS Google Scholar
Burton BK, Kronn DF, Hwu WL, Kishnani PS, Pompe Disease Newborn Screening Working G. The Initial Evaluation of Patients After Positive Newborn Screening: Recommended Algorithms Leading to a Confirmed Diagnosis of Pompe Disease. Pediatrics 2017;140(Suppl 1):S14-S23. https://doi.org/10.1542/peds.2016-0280D .
Griffin JL. Infantile acid maltase deficiency. I. Muscle fiber destruction after lysosomal rupture4. Virchows Arch Cell Pathol Mol Pathol 1984;45(1):23–36.
Thurberg BL, Lynch MC, Vaccaro C, et al. Characterization of pre- and post-treatment pathology after enzyme replacement therapy for pompe disease. Lab Investig 2006;86(12):1208–1220.
Lieberman AP, Puertollano R, Raben N, Slaugenhaupt S, Walkley SU, Ballabio A. Autophagy in lysosomal storage disorders. Autophagy 2012;8(5):719–730. https://doi.org/10.4161/auto.19469 .
Klionsky DJ. Autophagy: from phenomenology to molecular understanding in less than a decade. Nat Rev Mol Cell Biol. 2007;8(11):931–937.
Li WW, Li J, Bao JK. Microautophagy: lesser-known self-eating. Cell Mol Life Sci 2012;69(7):1125–1136. https://doi.org/10.1007/s00018-011-0865-5 .
Kaushik S, Bandyopadhyay U, Sridhar S, et al. Chaperone-mediated autophagy at a glance. J Cell Sci 2011;124(Pt 4):495–499. https://doi.org/10.1242/jcs.073874 .
Yang Z, Klionsky DJ. Mammalian autophagy: core molecular machinery and signaling regulation. Curr Opin Cell Biol 2010;22(2):124–131. https://doi.org/10.1016/j.ceb.2009.11.014 .
Klionsky DJ, Abdelmohsen K, Abe A, et al. Guidelines for the use and interpretation of assays for monitoring autophagy (3rd edition). Autophagy 2016;12(1):1–222. https://doi.org/10.1080/15548627.2015.1100356 .
Kabeya Y, Mizushima N, Ueno T, et al. LC3, a mammalian homologue of yeast Apg8p, is localized in autophagosome membranes after processing. EMBO J 2000;19(21):5720–5728.
Engel AG. Acid maltase deficiency in adults: studies in four cases of a syndrome which may mimic muscular dystrophy or other myopathies. Brain 1970;93(3):599–616.
Fukuda T, Ahearn M, Roberts A, et al. Autophagy and mistargeting of therapeutic enzyme in skeletal muscle in pompe disease. Mol Ther 2006;14(6):831–839.
Raben N, Baum R, Schreiner C, et al. When more is less: excess and deficiency of autophagy coexist in skeletal muscle in Pompe disease. Autophagy 2009;5(1):111–113.
Raben N, Takikita S, Pittis MG, et al. Deconstructing Pompe disease by analyzing single muscle fibers. Autophagy 2007;3(6):546–552.
Nishino I. Autophagic vacuolar myopathies. Curr Neurol Neurosci Rep 2003;3(1):64–9.
Spampanato C, Feeney E, Li L, et al. Transcription factor EB (TFEB) is a new therapeutic target for Pompe disease. EMBO Mol Med 2013;5:691–706. https://doi.org/10.1002/emmm.201202176 .
Nascimbeni AC, Fanin M, Tasca E, Angelini C, Sandri M. Impaired autophagy affects acid alpha-glucosidase processing and enzyme replacement therapy efficacy in late-onset glycogen storage disease type II. Neuropathol Appl Neurobiol 2015. https://doi.org/10.1111/nan.12214 .
Youle RJ, Narendra DP. Mechanisms of mitophagy. Nat Rev Mol Cell Biol 2011;12(1):9–14. https://doi.org/10.1038/nrm3028 .
Schoser BG, Muller-Hocker J, Horvath R, et al. Adult-onset glycogen storage disease type 2: clinico-pathological phenotype revisited. Neuropathol Appl Neurobiol 2007;33(5):544–559.
Lim JA, Li L, Kakhlon O, Myerowitz R, Raben N. Defects in calcium homeostasis and mitochondria can be reversed in Pompe disease. Autophagy 2015;11(2):385–402. https://doi.org/10.1080/15548627.2015.1009779 .
Ishigaki K, Mitsuhashi S, Kuwatsuru R, et al. High-density areas on muscle CT in childhood-onset Pompe disease are caused by excess calcium accumulation. Acta Neuropathol 2010;120(4):537–543. https://doi.org/10.1007/s00401-010-0732-8 .
Drost MR, Hesselink RP, Oomens CW, van der Vusse GJ. Effects of non-contractile inclusions on mechanical performance of skeletal muscle. J Biomech 2005;38(5):1035–1043.
Lim JA, Li L, Raben N. Pompe disease: from pathophysiology to therapy and back again. Front Aging Neurosci 2014;6:177. https://doi.org/10.3389/fnagi.2014.00177 .
Bar-Peled L, Sabatini DM. Regulation of mTORC1 by amino acids. Trends Cell Biol 2014. https://doi.org/10.1016/j.tcb.2014.03.003 .
Yoon MS. mTOR as a key regulator in maintaining skeletal muscle mass. Front Physiol 2017;8:788. https://doi.org/10.3389/fphys.2017.00788 .
Lim JA, Li L, Shirihai OS, Trudeau KM, Puertollano R, Raben N. Modulation of mTOR signaling as a strategy for the treatment of Pompe disease. EMBO Mol Med. 2017. https://doi.org/10.15252/emmm.201606547 .
Fratantoni JC, Hall CW, Neufeld EF. Hurler and Hunter syndromes: mutual correction of the defect in cultured fibroblasts. Science 1968;162(3853):570–572.
Neufeld EF. From serendipity to therapy. Annu Rev Biochem 2011;80:1–15. https://doi.org/10.1146/annurev.biochem.031209.093756 .
Dahms NM, Lobel P, Kornfeld S. Mannose 6-phosphate receptors and lysosomal enzyme targeting. J Biol Chem. 1989;264(21):12115–12118.
Van den Hout H, Reuser AJ, Vulto AG, Loonen MC, Cromme-Dijkhuis A, Van der Ploeg AT. Recombinant human alpha-glucosidase from rabbit milk in Pompe patients. Lancet 2000;356(9227):397–398.
Van den Hout JM, Kamphoven JH, Winkel LP, et al. Long-term intravenous treatment of Pompe disease with recombinant human alpha-glucosidase from milk. Pediatrics 2004;113(5):e448-e57.
Amalfitano A, Bengur AR, Morse RP, et al. Recombinant human acid alpha-glucosidase enzyme therapy for infantile glycogen storage disease type II: results of a phase I/II clinical trial. Genet Med 2001;3(2):132–138.
Kishnani PS, Corzo D, Nicolino M, et al. Recombinant human acid [alpha]-glucosidase: major clinical benefits in infantile-onset Pompe disease. Neurology 2007;68(2):99–109.
Kishnani PS, Corzo D, Leslie ND, et al. Early treatment with alglucosidase alpha prolongs long-term survival of infants with Pompe disease. Pediatr Res 2009;66(3):329–335.
Nicolino M, Byrne B, Wraith JE, et al. Clinical outcomes after long-term treatment with alglucosidase alfa in infants and children with advanced Pompe disease. Genet Med 2009;11(3):210–219.
Chakrapani A, Vellodi A, Robinson P, Jones S, Wraith JE. Treatment of infantile Pompe disease with alglucosidase alpha: the UK experience. J Inherit Metab Dis 2010;33(6):747–750. https://doi.org/10.1007/s10545-010-9206-3 .
Hahn A, Praetorius S, Karabul N, et al. Outcome of patients with classical infantile pompe disease receiving enzyme replacement therapy in Germany. JIMD Reports 2015. https://doi.org/10.1007/8904_2014_392 .
van Gelder CM, van Capelle CI, Ebbink BJ, et al. Facial-muscle weakness, speech disorders and dysphagia are common in patients with classic infantile Pompe disease treated with enzyme therapy. J Inherit Metab Dis 2012;35(3):505–511. https://doi.org/10.1007/s10545-011-9404-7 .
Prater SN, Banugaria SG, DeArmey SM, et al. The emerging phenotype of long-term survivors with infantile Pompe disease. Genet Med 2012;14(9):800–810. https://doi.org/10.1038/gim.2012.44 .
van Gelder CM, Poelman E, Plug I, et al. Effects of a higher dose of alglucosidase alfa on ventilator-free survival and motor outcome in classic infantile Pompe disease: an open-label single-center study. J Inherit Metab Dis 2016;39(3):383–390. https://doi.org/10.1007/s10545-015-9912-y .
Ebbink BJ, Poelman E, Aarsen FK, et al. Classic infantile Pompe patients approaching adulthood: a cohort study on consequences for the brain. Dev Med Child Neurol 2018;60(6):579–586. https://doi.org/10.1111/dmcn.13740 .
Banugaria SG, Prater SN, Ng YK, et al. The impact of antibodies on clinical outcomes in diseases treated with therapeutic protein: lessons learned from infantile Pompe disease. Genet Med 2011;13(8):729–736. https://doi.org/10.1097/GIM.0b013e3182174703 .
van Gelder CM, Hoogeveen-Westerveld M, Kroos MA, Plug I, van der Ploeg AT, Reuser AJ. Enzyme therapy and immune response in relation to CRIM status: the Dutch experience in classic infantile Pompe disease. J Inherit Metab Dis 2015;38(2):305–314. https://doi.org/10.1007/s10545-014-9707-6 .
Kishnani PS, Goldenberg PC, DeArmey SL, et al. Cross-reactive immunologic material status affects treatment outcomes in Pompe disease infants. Mol Genet Metab 2010;99(1):26–33.
Messinger YH, Mendelsohn NJ, Rhead W, et al. Successful immune tolerance induction to enzyme replacement therapy in CRIM-negative infantile Pompe disease. Genet Med 2012;14(1):135–142. https://doi.org/10.1038/gim.2011.4 .
Banugaria SG, Prater SN, Patel TT, et al. Algorithm for the early diagnosis and treatment of patients with cross reactive immunologic material-negative classic infantile pompe disease: a step towards improving the efficacy of ERT. PLoS One 2013;8(6):e67052. https://doi.org/10.1371/journal.pone.0067052 .
Banugaria SG, Prater SN, McGann JK, et al. Bortezomib in the rapid reduction of high sustained antibody titers in disorders treated with therapeutic protein: lessons learned from Pompe disease. Genet Med 2013;15(2):123–131. https://doi.org/10.1038/gim.2012.110 .
de Vries JM, van der Beek NA, Kroos MA, et al. High antibody titer in an adult with Pompe disease affects treatment with alglucosidase alfa. Mol Genet Metab 2010;101(4):338–345. https://doi.org/10.1016/j.ymgme.2010.08.009 .
Patel TT, Banugaria SG, Case LE, Wenninger S, Schoser B, Kishnani PS. The impact of antibodies in late-onset Pompe disease: a case series and literature review. Mol Genet Metab 2012;106(3):301–319. https://doi.org/10.1016/j.ymgme.2012.04.027 .
Kronn DF, Day-Salvatore D, Hwu WL, et al. Management of confirmed newborn-screened patients with Pompe disease across the disease spectrum. Pediatrics 2017;140(Suppl 1):S24-S45. https://doi.org/10.1542/peds.2016-0280E .
Van der Ploeg AT, Clemens PR, Corzo D, et al. A randomized study of alglucosidase alfa in late-onset Pompe's disease. N Engl J Med 2010;362(15):1396–1406.
Wokke JH, Escolar DM, Pestronk A, et al. Clinical features of late-onset Pompe disease: a prospective cohort study. Muscle Nerve 2008;38(4):1236–1245. https://doi.org/10.1002/mus.21025 .
van der Ploeg AT, Barohn R, Carlson L, et al. Open-label extension study following the Late-Onset Treatment Study (LOTS) of alglucosidase alfa. Mol Genet Metab 2012;107(3):456–461. https://doi.org/10.1016/j.ymgme.2012.09.015 .
Strothotte S, Strigl-Pill N, Grunert B, et al. Enzyme replacement therapy with alglucosidase alfa in 44 patients with late-onset glycogen storage disease type 2: 12-month results of an observational clinical trial. J Neurol 2010;257(1):91–97. https://doi.org/10.1007/s00415-009-5275-3 .
van Capelle CI, van der Beek NA, Hagemans ML, et al. Effect of enzyme therapy in juvenile patients with Pompe disease: a three-year open-label study. Neuromuscul Disord 2010;20(12):775–782. https://doi.org/10.1016/j.nmd.2010.07.277 .
Bembi B, Pisa FE, Confalonieri M, et al. Long-term observational, non-randomized study of enzyme replacement therapy in late-onset glycogenosis type II. J Inherit Metab Dis 2010;33(6):727–735. https://doi.org/10.1007/s10545-010-9201-8 .
Angelini C, Semplicini C, Ravaglia S, et al. Observational clinical study in juvenile-adult glycogenosis type 2 patients undergoing enzyme replacement therapy for up to 4 years. J Neurol 2012;259(5):952–958. https://doi.org/10.1007/s00415-011-6293-5 .
Toscano A, Schoser B. Enzyme replacement therapy in late-onset Pompe disease: a systematic literature review. J Neurol 2013;260(4):951–959. https://doi.org/10.1007/s00415-012-6636-x .
Schoser B, Stewart A, Kanters S, et al. Survival and long-term outcomes in late-onset Pompe disease following alglucosidase alfa treatment: a systematic review and meta-analysis. J Neurol 2017;264(4):621–630. https://doi.org/10.1007/s00415-016-8219-8 .
Anderson LJ, Henley W, Wyatt KM, et al. Effectiveness of enzyme replacement therapy in adults with late-onset Pompe disease: results from the NCS-LSD cohort study. J Inherit Metab Dis 2014;37(6):945–52. https://doi.org/10.1007/s10545-014-9728-1 .
Gungor D, de Vries JM, Brusse E, et al. Enzyme replacement therapy and fatigue in adults with Pompe disease. Mol Genet Metab 2013;109(2):174–178. https://doi.org/10.1016/j.ymgme.2013.03.016 .
Gungor D, Kruijshaar ME, Plug I, et al. Impact of enzyme replacement therapy on survival in adults with Pompe disease: results from a prospective international observational study. Orphanet J Rare Dis. 2013;8:49. https://doi.org/10.1186/1750-1172-8-49 .
Wenk J, Hille A, von Figura K. Quantitation of Mr 46000 and Mr 300000 mannose 6-phosphate receptors in human cells and tissues. Biochem Int 1991;23(4):723–731.
Zhu Y, Jiang JL, Gumlaw NK, et al. Glycoengineered acid alpha-glucosidase with improved efficacy at correcting the metabolic aberrations and motor function deficits in a mouse model of Pompe disease. Mol Ther 2009;17(6):954–963.
Ghosh P, Dahms NM, Kornfeld S. Mannose 6-phosphate receptors: new twists in the tale. Nat Rev Mol Cell Biol. 2003;4(3):202–212.
LeBowitz JH, Grubb JH, Maga JA, Schmiel DH, Vogler C, Sly WS. Glycosylation-independent targeting enhances enzyme delivery to lysosomes and decreases storage in mucopolysaccharidosis type VII mice. Proc Natl Acad Sci U S A 2004;101(9):3083–3088. https://doi.org/10.1073/pnas.0308728100 .
Maga JA, Zhou J, Kambampati R, et al. Glycosylation-independent lysosomal targeting of acid alpha-glucosidase enhances muscle glycogen clearance in Pompe mice. J Biol Chem 2013;288:1428–1438. https://doi.org/10.1074/jbc.M112.438663 .
Basile I, Da Silva A, El Cheikh K, et al. Efficient therapy for refractory Pompe disease by mannose 6-phosphate analogue grafting on acid alpha-glucosidase. J Control Release 2018;269:15–23. https://doi.org/10.1016/j.jconrel.2017.10.043 .
Kang JY, Shin KK, Kim HH, et al. Lysosomal targeting enhancement by conjugation of glycopeptides containing mannose-6-phosphate glycans derived from glyco-engineered yeast. Sci Rep 2018;8(1):8730. https://doi.org/10.1038/s41598-018-26913-4 .
Koeberl DD, Luo X, Sun B, et al. Enhanced efficacy of enzyme replacement therapy in Pompe disease through mannose-6-phosphate receptor expression in skeletal muscle. Mol Genet Metab 2011;103(2):107–112. https://doi.org/10.1016/j.ymgme.2011.02.006 .
Koeberl DD, Li S, Dai J, Thurberg BL, Bali D, Kishnani PS. beta2 Agonists enhance the efficacy of simultaneous enzyme replacement therapy in murine Pompe disease. Mol Genet Metab 2012;105(2):221–227. https://doi.org/10.1016/j.ymgme.2011.11.005 .
Koeberl DD, Austin S, Case LE, et al. Adjunctive albuterol enhances the response to enzyme replacement therapy in late-onset Pompe disease. FASEB J 2014;28(5):2171–2176. https://doi.org/10.1096/fj.13-241893 .
Parenti G, Moracci M, Fecarotta S, Andria G. Pharmacological chaperone therapy for lysosomal storage diseases. Future Med Chem 2014;6(9):1031–1045. https://doi.org/10.4155/fmc.14.40 .
Parenti G, Fecarotta S, la Marca G, et al. A chaperone enhances blood alpha-glucosidase activity in Pompe disease patients treated with enzyme replacement therapy. Mol Ther 2014;22(11):2004–2012. https://doi.org/10.1038/mt.2014.138 .
Douillard-Guilloux G, Raben N, Takikita S, Batista L, Caillaud C, Richard E. Modulation of glycogen synthesis by RNA interference: towards a new therapeutic approach for glycogenosis type II. Hum Mol Genet 2008;17(24):3876–3886.
Douillard-Guilloux G, Raben N, Takikita S, et al. Restoration of muscle functionality by genetic suppression of glycogen synthesis in a murine model of Pompe disease. Hum Mol Genet 2010;19(4):684–696. https://doi.org/10.1093/hmg/ddp535 .
Raben N, Schreiner C, Baum R, et al. Suppression of autophagy permits successful enzyme replacement therapy in a lysosomal storage disorder-murine Pompe disease. Autophagy 2010;6(8):1078–1089.
Andrews NW. Regulated secretion of conventional lysosomes. Trends Cell Biol 2000;10(8):316–321.
Settembre C, Ballabio A. Lysosomal adaptation: how the lysosome responds to external cues. Cold Spring Harb Perspect Biol 2014. https://doi.org/10.1101/cshperspect.a016907 .
Sardiello M, Palmieri M, di Ronza A, et al. A gene network regulating lysosomal biogenesis and function. Science 2009;325(5939):473–477. https://doi.org/10.1126/science.1174447 .
Medina DL, Fraldi A, Bouche V, et al. Transcriptional activation of lysosomal exocytosis promotes cellular clearance. Dev Cell 2011;21(3):421–430. https://doi.org/10.1016/j.devcel.2011.07.016 .
Martina JA, Diab HI, Li L, et al. the nutrient-responsive transcription factor TFE3 Promotes autophagy, lysosomal biogenesis, and clearance of cellular debris. Sci Signal. 2014;7(309):ra9. https://doi.org/10.1126/scisignal.2004754 .
Gatto F, Rossi B, Tarallo A, et al. AAV-mediated transcription factor EB (TFEB) gene delivery ameliorates muscle pathology and function in the murine model of Pompe Disease. Sci Rep 2017;7(1):15089. https://doi.org/10.1038/s41598-017-15352-2 .
Zaretsky JZ, Candotti F, Boerkoel C, et al. Retroviral transfer of acid alpha-glucosidase cDNA to enzyme-deficient myoblasts results in phenotypic spread of the genotypic correction by both secretion and fusion. Hum Gene Ther 1997;8(13):1555–1563.
Pauly DF, Johns DC, Matelis LA, Lawrence JH, Byrne BJ, Kessler PD. Complete correction of acid alpha-glucosidase deficiency in Pompe disease fibroblasts in vitro, and lysosomally targeted expression in neonatal rat cardiac and skeletal muscle. Gene Ther 1998;5(4):473–480.
Amalfitano A, McVie-Wylie AJ, Hu H, et al. Systemic correction of the muscle disorder glycogen storage disease type II after hepatic targeting of a modified adenovirus vector encoding human acid-alpha-glucosidase. Proc Natl Acad Sci U S A 1999;96(16):8861–8866.
Fraites TJ, Jr, Schleissing MR, Shanely RA, et al. Correction of the enzymatic and functional deficits in a model of Pompe disease using adeno-associated virus vectors. Mol Ther 2002;5(5 Pt 1):571–578.
Richard E, Douillard-Guilloux G, Batista L, Caillaud C. Correction of glycogenosis type 2 by muscle-specific lentiviral vector. In Vitro Cell Dev Biol Anim 2008;44(10):397–406. https://doi.org/10.1007/s11626-008-9138-5 .
Sato Y, Kobayashi H, Higuchi T, et al. Disease modeling and lentiviral gene transfer in patient-specific induced pluripotent stem cells from late-onset Pompe disease patient. Mol Ther Methods Clin Dev 2015;2:15023. https://doi.org/10.1038/mtm.2015.23 .
Kyosen SO, Iizuka S, Kobayashi H, et al. Neonatal gene transfer using lentiviral vector for murine Pompe disease: long-term expression and glycogen reduction. Gene Ther 2010;17(4):521–530. https://doi.org/10.1038/gt.2009.160 .
Athanasopoulos T, Munye MM, Yanez-Munoz RJ. Nonintegrating gene therapy vectors. Hematol Oncol Clin North Am 2017;31(5):753–770. https://doi.org/10.1016/j.hoc.2017.06.007 .
Sun B, Zhang H, Franco LM, et al. Correction of glycogen storage disease type II by an adeno-associated virus vector containing a muscle-specific promoter. Mol Ther 2005;11(6):889–898.
DeRuisseau LR, Fuller DD, Qiu K, et al. Neural deficits contribute to respiratory insufficiency in Pompe disease. Proc Natl Acad Sci U S A 2009;106(23):9419–9424. https://doi.org/10.1073/pnas.0902534106 .
Fuller DD, ElMallah MK, Smith BK, et al. The respiratory neuromuscular system in Pompe disease. Respir Physiol Neurobiol 2013;189(2):241–249. https://doi.org/10.1016/j.resp.2013.06.007 .
Todd AG, McElroy JA, Grange RW, et al. Correcting neuromuscular deficits with gene therapy in Pompe disease. Ann Neurol 2015. https://doi.org/10.1002/ana.24433 .
Qiu K, Falk DJ, Reier PJ, Byrne BJ, Fuller DD. Spinal delivery of AAV vector restores enzyme activity and increases ventilation in Pompe mice. Mol Ther 2012;20(1):21–27. https://doi.org/10.1038/mt.2011.214 .
Hordeaux J, Dubreil L, Robveille C, et al. Long-term neurologic and cardiac correction by intrathecal gene therapy in Pompe disease. Acta Neuropathologica Communications 2017;5(1):66. https://doi.org/10.1186/s40478-017-0464-2 .
Lee NC, Hwu WL, Muramatsu SI, et al. A neuron-specific gene therapy relieves motor deficits in pompe disease mice. Mol Neurobiol 2017. https://doi.org/10.1007/s12035-017-0763-4 .
Corti M, Elder M, Falk D, et al. B-cell depletion is protective against anti-AAV capsid immune response: a human subject case study. Mol Ther Methods Clin Dev 2014;1. https://doi.org/10.1038/mtm.2014.33 .
Byrne BJ, Falk DJ, Pacak CA, et al. Pompe disease gene therapy. Hum Mol Genet 2011;20(R1):R61–R68. https://doi.org/10.1093/hmg/ddr174 .
Doerfler PA, Nayak S, Corti M, Morel L, Herzog RW, Byrne BJ. Targeted approaches to induce immune tolerance for Pompe disease therapy. Mol Ther Methods Clin Dev 2016;3:15053. https://doi.org/10.1038/mtm.2015.53 .
Bond JE, Kishnani PS, Koeberl DD. Immunomodulatory, liver depot gene therapy for Pompe disease. Cell Immunol 2017. https://doi.org/10.1016/j.cellimm.2017.12.011 .
Mah C, Pacak CA, Cresawn KO, et al. Physiological correction of Pompe disease by systemic delivery of adeno-associated virus serotype 1 vectors. Mol Ther 2007;15(3):501–507.
Mah CS, Falk DJ, Germain SA, et al. Gel-mediated delivery of AAV1 vectors corrects ventilatory function in Pompe mice with established disease. Mol Ther 2010;18(3):502–510. https://doi.org/10.1038/mt.2009.305 .
Elmallah MK, Falk DJ, Nayak S, et al. Sustained correction of motoneuron histopathology following intramuscular delivery of AAV in pompe mice. Mol Ther 2014;22(4):702–712. https://doi.org/10.1038/mt.2013.282 .
Smith BK, Collins SW, Conlon TJ, et al. Phase I/II trial of adeno-associated virus-mediated alpha-glucosidase gene therapy to the diaphragm for chronic respiratory failure in Pompe disease: initial safety and ventilatory outcomes. Hum Gene Ther 2013;24(6):630–640. https://doi.org/10.1089/hum.2012.250 .
Byrne PI, Collins S, Mah CC, et al. Phase I/II trial of diaphragm delivery of recombinant adeno-associated virus acid alpha-glucosidase (rAAaV1-CMV-GAA) gene vector in patients with Pompe disease. Hum Gene Ther Clin Dev 2014;25(3):134–163. https://doi.org/10.1089/humc.2014.2514 .
Smith BK, Martin AD, Lawson LA, et al. Inspiratory muscle conditioning exercise and diaphragm gene therapy in Pompe disease: Clinical evidence of respiratory plasticity. Exp Neurol 2017;287(Pt 2):216–224. https://doi.org/10.1016/j.expneurol.2016.07.013 .
Corti M, Liberati C, Smith BK, et al. Safety of intradiaphragmatic delivery of adeno-associated virus-mediated alpha-glucosidase (rAAV1-CMV-hGAA) gene therapy in children affected by pompe disease. Hum Gene Ther Clin Dev 2017;28(4):208–218. https://doi.org/10.1089/humc.2017.146 .
Falk DJ, Mah CS, Soustek MS, et al. Intrapleural administration of AAV9 improves neural and cardiorespiratory function in Pompe disease. Mol Ther 2013;21(9):1661–1667. https://doi.org/10.1038/mt.2013.96 .
Falk DJ, Soustek MS, Todd AG, et al. Comparative impact of AAV and enzyme replacement therapy on respiratory and cardiac function in adult Pompe mice. Mol Ther Methods Clin Dev 2015;2:15007. https://doi.org/10.1038/mtm.2015.7 .
Corti M, Cleaver B, Clement N, et al. Evaluation of readministration of a recombinant adeno-associated virus vector expressing acid alpha-glucosidase in pompe disease: preclinical to clinical planning. Hum Gene Ther Clin Dev 2015;26(3):185–193. https://doi.org/10.1089/humc.2015.068 .
Sun B, Kulis MD, Young SP, et al. Immunomodulatory gene therapy prevents antibody formation and lethal hypersensitivity reactions in murine pompe disease. Mol Ther 2010;18(2):353–360. https://doi.org/10.1038/mt.2009.195 .
Han SO, Ronzitti G, Arnson B, et al. Low-dose liver-targeted gene therapy for pompe disease enhances therapeutic efficacy of ERT via immune tolerance induction. Mol Ther Methods Clin Dev 2017;4:126–136. https://doi.org/10.1016/j.omtm.2016.12.010 .
Puzzo F, Colella P, Biferi MG, et al. Rescue of Pompe disease in mice by AAV-mediated liver delivery of secretable acid alpha-glucosidase. Sci Transl Med. 2017;9(418). https://doi.org/10.1126/scitranslmed.aam6375 .
Fu H, Dirosario J, Killedar S, Zaraspe K, McCarty DM. Correction of neurological disease of mucopolysaccharidosis IIIB in adult mice by rAAV9 trans-blood-brain barrier gene delivery. Mol Ther 2011;19(6):1025–1033. https://doi.org/10.1038/mt.2011.34 .
Wang H, La Russa M, Qi LS. CRISPR/Cas9 in Genome Editing and Beyond. Annu Rev Biochem 2016;85:227–264. https://doi.org/10.1146/annurev-biochem-060815-014607 .
Long C, Amoasii L, Mireault AA, et al. Postnatal genome editing partially restores dystrophin expression in a mouse model of muscular dystrophy. Science 2016;351(6271):400–403. https://doi.org/10.1126/science.aad5725 .
Nelson CE, Hakim CH, Ousterout DG, et al. In vivo genome editing improves muscle function in a mouse model of Duchenne muscular dystrophy. Science 2016;351(6271):403–407. https://doi.org/10.1126/science.aad5143 .
Xu L, Park KH, Zhao L, et al. CRISPR-mediated genome editing restores dystrophin expression and function in mdx mice. Mol Ther 2016;24(3):564–569. https://doi.org/10.1038/mt.2015.192 .
Bengtsson NE, Hall JK, Odom GL, et al. Muscle-specific CRISPR/Cas9 dystrophin gene editing ameliorates pathophysiology in a mouse model for Duchenne muscular dystrophy. Nat Commun 2017;8:14454. https://doi.org/10.1038/ncomms14454 .
Kemaladewi DU, Maino E, Hyatt E, et al. Correction of a splicing defect in a mouse model of congenital muscular dystrophy type 1A using a homology-directed-repair-independent mechanism. Nat Med 2017;23(8):984–989. https://doi.org/10.1038/nm.4367 .
Suzuki K, Tsunekawa Y, Hernandez-Benitez R, et al. In vivo genome editing via CRISPR/Cas9 mediated homology-independent targeted integration. Nature 2016;540(7631):144–149. https://doi.org/10.1038/nature20565 .
Chien YH, Chiang SC, Zhang XK, et al. Early detection of Pompe disease by newborn screening is feasible: results from the Taiwan screening program. Pediatrics 2008;122(1):e39-e45.
Chien YH, Lee NC, Thurberg BL, et al. Pompe disease in infants: improving the prognosis by newborn screening and early treatment. Pediatrics 2009;124(6):e1116-e1125.
Chien YH, Hwu WL, Lee NC. Pompe disease: early diagnosis and early treatment make a difference. Pediatr Neonatol 2013. https://doi.org/10.1016/j.pedneo.2013.03.009 .
Yang CF, Liu HC, Hsu TR, et al. A large-scale nationwide newborn screening program for Pompe disease in Taiwan: towards effective diagnosis and treatment. Am J Med Genet A 2014;164A(1):54–61. https://doi.org/10.1002/ajmg.a.36197 .
Martiniuk F, Chen A, Mack A, et al. Carrier frequency for glycogen storage disease type II in New York and estimates of affected individuals born with the disease. Am J Med Genet 1998;79(1):69–72.
Ausems MG, Verbiest J, Hermans MP, et al. Frequency of glycogen storage disease type II in The Netherlands: implications for diagnosis and genetic counselling. Eur J Hum Genet 1999;7(6):713–716.
Yang CC, Chien YH, Lee NC, et al. Rapid progressive course of later-onset Pompe disease in Chinese patients. Mol Genet Metab 2011;104(3):284–288. https://doi.org/10.1016/j.ymgme.2011.06.010 .
Chien YH, Lee NC, Huang HJ, Thurberg BL, Tsai FJ, Hwu WL. Later-onset Pompe disease: early detection and early treatment initiation enabled by newborn screening. J Pediatr 2011;158(6):1023–1027 e1. https://doi.org/10.1016/j.jpeds.2010.11.053 .
Kishnani PS, Amartino HM, Lindberg C, et al. Timing of diagnosis of patients with Pompe disease: data from the Pompe registry. Am J Med Genet A 2013;161A(10):2431–2443. https://doi.org/10.1002/ajmg.a.36110 .
Rairikar MV, Case LE, Bailey LA, et al. Insight into the phenotype of infants with Pompe disease identified by newborn screening with the common c.-32-13T>G “late-onset” GAA variant. Mol Genet Metab 2017;122(3):99–107. https://doi.org/10.1016/j.ymgme.2017.09.008 .
Chamoles NA, Niizawa G, Blanco M, Gaggioli D, Casentini C. Glycogen storage disease type II: enzymatic screening in dried blood spots on filter paper. Clin Chim Acta 2004;347(1–2):97–102.
Bodamer OA, Scott CR, Giugliani R, Pompe Disease Newborn Screening Working G. Newborn screening for pompe disease Pediatrics 2017;140(Suppl 1):S4-S13. https://doi.org/10.1542/peds.2016-0280C .
Download references
Acknowledgments
We apologize to all colleagues whose publications were not cited because of space limitations.
This research was supported in part by the Intramural Research Program of the National Heart, Lung, and Blood Institute, National Institutes of Health. Dr. Kohler is supported in part by a CRADA between NIH and Genzyme Corporation and by the Acid Maltase Deficiency Association.
Author information
Authors and affiliations.
Cell Biology and Physiology Center, National Heart, Lung, and Blood Institute, National Institutes of Health, Bethesda, MD, USA
Lara Kohler, Rosa Puertollano & Nina Raben
You can also search for this author in PubMed Google Scholar
Corresponding authors
Correspondence to Rosa Puertollano or Nina Raben .
Ethics declarations
Required author forms.
Disclosure forms provided by the authors are available with the version of this article.
Conflict of Interest
The authors declare that they have no conflict of interest.
Electronic Supplementary Material
(PDF 1196 kb)
Rights and permissions
Reprints and permissions
About this article
Kohler, L., Puertollano, R. & Raben, N. Pompe Disease: From Basic Science to Therapy. Neurotherapeutics 15 , 928–942 (2018). https://doi.org/10.1007/s13311-018-0655-y
Download citation
Published : 16 August 2018
Issue Date : 14 October 2018
DOI : https://doi.org/10.1007/s13311-018-0655-y
Share this article
Anyone you share the following link with will be able to read this content:
Sorry, a shareable link is not currently available for this article.
Provided by the Springer Nature SharedIt content-sharing initiative
- Glycogen storage
- enzyme replacement therapy
- newborn screening
- Find a journal
- Publish with us
- Track your research
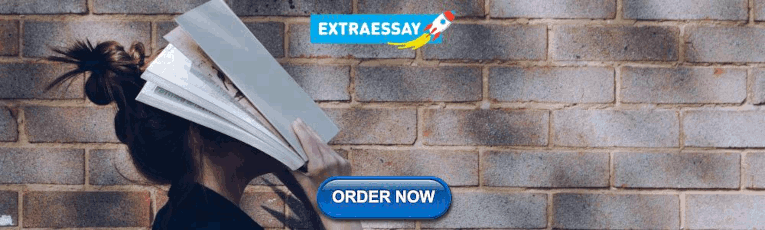
Pompe disease ascertained through The Lantern Project, 2018-2021: Next-generation sequencing and enzymatic testing to overcome obstacles to diagnosis
Affiliations.
- 1 Sanofi, Cambridge, MA, USA. Electronic address: [email protected].
- 2 PerkinElmer Genomics, Pittsburgh, PA, USA.
- 3 Sanofi, Cambridge, MA, USA.
- PMID: 37087815
- DOI: 10.1016/j.ymgme.2023.107565
The Lantern Project is an ongoing complimentary diagnostic program for patients in the United States sponsored by Sanofi and implemented by PerkinElmer Genomics. It combines specific enzymatic, biomarker, and genetic testing to facilitate rapid, accurate laboratory diagnosis of Pompe disease and several other lysosomal storage diseases, and a multigene next-generation sequencing panel including Pompe disease, LGMD, and other neuromuscular disorders. This article reports data for Pompe disease collected from October 2018 through December 2021, including acid α-glucosidase (GAA) enzyme assay and GAA sequencing (standard or expedited for positive newborn screening [NBS] to rule out infantile-onset Pompe disease [IOPD]) and the Focused Neuromuscular Panel, which includes GAA. One hundred forty patients (12 received only GAA enzyme testing, 128 had GAA sequencing alone or in addition to enzyme assay) have been confirmed with Pompe disease in this project. Eight of the 140 had a variant of unknown significance, but GAA activity ≤2.10 μmol/L/h, thus were confirmed with Pompe disease. Three diagnosed patients 0-2 years old had cross-reactive immunologic material (CRIM)-negative GAA variants and thus IOPD. One additional infant with presumptive IOPD had a homozygous frameshift c.1846del, likely CRIM-negative; symptoms were not provided. Among the 128 patients with molecular results, the c.-32-13T>G splice variant was homozygous in 11, compound-heterozygous in 98, and absent in 19. Proximal muscle weakness (58 patients) was the most common sign reported at testing; elevated creatine kinase (29 patients) was the most common laboratory result. The most common symptom categories were muscular (73 patients), musculoskeletal (13 patients), and respiratory (23 patients). Clinical information was not available for 42 samples, and 17 infants had only "abnormal NBS" or "low GAA" reported. Cardiac symptoms in 7 included potentially age-related conditions in five c.-32-13T>G-compound-heterozygous adults (myocardial infarction, heart murmur/palpitations, congestive heart failure: 1 each; 2 with atrial fibrillation) and hypertrophic cardiomyopathy in 2 children (1 and 2 years old) with presumptive IOPD. One novel GAA variant was observed in a patient with enzyme activity 0.31 μmol/L/h: c.1853_1854ins49, a frameshift pathogenic variant. The Lantern Project demonstrates the combinatorial utility of enzyme assay, targeted single-gene testing, and a focused neuromuscular next-generation sequencing panel in diagnosing Pompe disease.
Keywords: Acid alpha-glucosidase; Dried blood spot; Limb-girdle muscle weakness; Newborn screening; Next-generation sequencing; Pompe disease.
Copyright © 2023. Published by Elsevier Inc.
Publication types
- Research Support, Non-U.S. Gov't
- Child, Preschool
- Glycogen Storage Disease Type II* / diagnosis
- Glycogen Storage Disease Type II* / genetics
- High-Throughput Nucleotide Sequencing / methods
- Infant, Newborn
- Neonatal Screening
- alpha-Glucosidases / genetics
- alpha-Glucosidases

Study could offer hope to Pompe disease patients
Researchers develop a new method that could improve enzyme replacement therapy in people with the deadly inherited disease..
Pompe disease is a rare genetic disorder that disables heart and skeletal muscles and can lead to early death if untreated. The only available treatment for the disease is enzyme replacement therapy that must be injected regularly, sometimes every few days, for life. The treatment can cost hundreds of thousands of dollars a year. Researchers from the University of Maryland developed a method that could make enzyme replacement therapy more efficient, less expensive and less frequent. A study on their new method was published on August 19, 2021, in the journal Chemical Science .
"This work holds great promise for a better, less costly treatment of the life-threatening Pompe disease," said UMD Chemistry and Biochemistry Professor Lai-Xi Wang, the corresponding author of the study.
In people with Pompe disease, the machinery inside their cells lacks a critical enzyme needed to turn glycogen into glucose, the sugar that fuels muscles. Instead of breaking down, glycogen builds up in the cells, eventually damaging muscle tissue, especially the heart.
Currently, the only treatment for Pompe disease involves injecting large amounts of the missing enzyme, known as acid alpha-glucosidase (GAA), into the bloodstream of patients -- with the hope that some of it will get into the cells and clean up the built-up glycogen. But, generally, only a small amount of the enzyme reaches the cellular machinery where it is needed.
"As a result of this inefficiency, a large quantity of the enzyme needs to be injected, and the cost can go up to a half-million dollars per patient a year," Wang said. "We have developed a simple method of modifying the enzyme that demonstrated much enhanced receptor binding, cellular uptake and glycogen degrading activity in cells.
To develop their method, Wang and his team studied the chemical synthesis and binding properties of various molecules and discovered a ligand -- a binding molecule -- that was easily recognized by a specific receptor in the cellular transport system. This system carries compounds from the bloodstream across the cell membrane into the machinery where glycogen is broken down into glucose.
Once they found that ligand, Wang's team developed a simple method to attach it to the GAA enzyme. Working with colleagues from the National Institutes of Health (NIH), the researchers tested their engineered GAA enzyme with the attached ligand in model cells and found that it was much more efficient at delivering GAA to the target machinery than the current enzyme replacement therapy.
"Our method could dramatically reduce the amount of enzyme and maybe also the frequency of injections needed to treat Pompe disease, because it ensures that more of the GAA enzyme gets into the cells' machinery where it can do its job," said Xiao Zhang, a postdoctoral associate in UMD's Department of Chemistry and Biochemistry and the lead author of the study
The research team is now doing further studies with collaborators at the NIH to evaluate the effects of the combined GAA-ligand therapy in animals with hopes of eventually moving to human trials in the future.
Pompe disease is one of about 50 diseases known as lysosome storage diseases in which cellular machinery lacks the enzymes needed to break down various waste products in cells. The researchers say the method they developed through this work could lead to improved treatments for other similar lysosome storage diseases.
- Diseases and Conditions
- Immune System
- Heart Disease
- Alzheimer's Research
- Pharmacology
- Hormone replacement therapy
- Coeliac disease
- Ischaemic heart disease
- Gene therapy
- Personalized medicine
- Physical therapy
- Heart failure
- Water purification
Story Source:
Materials provided by University of Maryland . Original written by Kimbra Cutlip. Note: Content may be edited for style and length.
Journal Reference :
- Xiao Zhang, Huiying Liu, Naresh Meena, Chao Li, Guanghui Zong, Nina Raben, Rosa Puertollano, Lai-Xi Wang. Chemoenzymatic glycan-selective remodeling of a therapeutic lysosomal enzyme with high-affinity M6P-glycan ligands. Enzyme substrate specificity is the name of the game . Chemical Science , 2021; DOI: 10.1039/D1SC03188K
Cite This Page :
Explore More
- Fossil Frogs Share Their Skincare Secrets
- Fussy Eater? Most Parents Play Short Order Cook
- Precise Time Measurement: Superradiant Atoms
- Artificial Cells That Act Like Living Cells
- Affordable and Targeted Anticancer Agent
- This Alloy Is Kinky
- Giant Galactic Explosion: Galaxy Pollution
- Flare Erupting Around a Black Hole
- Two Species Interbreeding Created New Butterfly
- Warming Antarctic Deep-Sea and Sea Level Rise
Trending Topics
Strange & offbeat.

An official website of the United States government
The .gov means it’s official. Federal government websites often end in .gov or .mil. Before sharing sensitive information, make sure you’re on a federal government site.
The site is secure. The https:// ensures that you are connecting to the official website and that any information you provide is encrypted and transmitted securely.
- Publications
- Account settings
Preview improvements coming to the PMC website in October 2024. Learn More or Try it out now .
- Advanced Search
- Journal List
- Pharmacoecon Open
- v.3(4); 2019 Dec
A Systematic Review of the Health Economics of Pompe Disease
Benedikt schoser.
1 Friedrich-Baur-Institut, Neurologische Klinik, Klinikum der Universität München, Ludwig-Maximilians-University Munich, Munich, Germany
Andreas Hahn
2 Department of Child Neurology, University of Giessen, Feulgenstrasse 12, 35385 Giessen, Germany
3 Audentes Therapeutics, 600 California Street, Floor 17, San Francisco, CA 94108 USA
Digant Gupta
4 Bridge Medical Consulting Ltd, Gainsborough House, 2 Sheen Road, Richmond, London, UK
Matthew Gitlin
5 BluePath Solutions, 10951 West Pico Blvd, Suite 120, Los Angeles, CA 90064 USA
Suyash Prasad
Associated data.
All data used in this literature review are derived from published studies and are thus already available.
Pompe disease is a rare, severe neuromuscular disease with high mortality and substantial clinical and humanistic burden. However, the economic burden of Pompe disease and the health economic value of its treatments are not well understood. The objectives of this systematic review were to characterize the health economic evidence on Pompe disease, including healthcare resource use and costs (direct and indirect), health utilities, and the cost-effectiveness of current treatments used to manage patients with Pompe disease.
A systematic search of MEDLINE ® and Embase ® was performed to retrieve publications on the health economics of Pompe disease. Publications were screened according to predefined criteria, extracted, and quality assessed using the Newcastle–Ottawa Scale. Data were narratively synthesized.
Eight publications evaluated patients with infantile-onset Pompe disease (IOPD) (two studies), late-onset Pompe disease (LOPD) (four studies), or both (two studies). In IOPD, total cost of supportive therapy (excluding treatment) was €32,871 (equivalent to US$41,667 when adjusted for currency and inflation to 2017 US dollars) over a life expectancy of 0.4 years. In adult LOPD, the average annual cost per patient of supportive therapy was €22,475 (adjusted $28,489). Resource use in LOPD was high, with nursing home admissions accounting for 19% of annual direct medical costs. Health economic evaluations estimating incremental costs per quality-adjusted life year (QALY) gained with enzyme-replacement therapy (ERT) versus supportive therapy ranged from £109,991 (adjusted, $186,851) per QALY gained in Columbia to €1,043,868 (adjusted, $1,323,207) in the Netherlands.
Despite a full systematic literature search, only eight relevant publications were identified, most of which were of relatively poor quality. However, a significant economic burden of Pompe disease on patients, families, healthcare systems, and society was found, with the majority of costs driven by the only currently approved treatment, ERT. Health economic evaluations of ERT versus supportive therapy vary significantly, with the majority well above willingness-to-pay thresholds. New therapies and approaches to care are needed to address the persistent and lifelong economic burden of Pompe disease and the large incremental cost-effectiveness ratios observed.
Electronic supplementary material
The online version of this article (10.1007/s41669-019-0142-3) contains supplementary material, which is available to authorized users.
Key Points for Decision Makers
By definition, rare diseases affect small numbers of individuals [no more than one in 2000 individuals in the European Union and one in 200,000 in the United States (US)] [ 1 ]. Costs per patient of treating rare diseases tend to be higher than those for more common conditions [ 2 – 4 ]. Pompe disease is a rare autosomal recessive, progressive, debilitating multisystemic neuromuscular disease in which acid α-glucosidase (GAA) deficiency leads to intralysosomal accumulations of glycogen in all tissues. Based on age at manifestation and severity of disease, two main types of Pompe disease are distinguished. At one end of the spectrum, infantile-onset Pompe disease (IOPD) usually presents with hypertrophic cardiomyopathy and skeletal muscle weakness within the first 6 months of life. Without treatment, most patients die within the first year of life without achieving any motor milestone such as turning, sitting, or walking [ 5 ]. At the other end, the more slowly progressing late-onset Pompe disease (LOPD) can manifest any time, from early childhood to adulthood, as skeletal myopathy without major cardiac involvement, although survival is still adversely affected [ 6 – 8 ].
Because of its low prevalence (estimated at one in 138,000 to one in 14,000 [ 9 – 11 ], but most commonly quoted at one in 40,000 [ 12 ]), Pompe disease is classified as an orphan disease. Although not unique to orphan therapies, orphan conditions like Pompe disease have been chronically under researched prior to the approval of the first drug treatment, enzyme-replacement therapy (ERT) with recombinant human GAA (rhGAA) [ 13 ]. The first and only ERTs with rhGAA for Pompe disease—Myozyme and Lumizyme (alglucosidase alfa)—were launched in Europe and the US in 2006 and 2010, respectively. Until 2014, Lumizyme was available in the US only to LOPD patients, before being granted a US license for all Pompe patients in line with the rest of the world [ 14 ]. The introduction of ERT for the management of Pompe disease has improved the prognosis for patients by reducing the mortality, progression, and burden of this serious disorder. However, ERT is far from a cure, with mortality remaining at around 28–43% in IOPD [ 15 – 18 ], and substantial morbidity persisting in most patients across the disease spectrum. Existing literature on the humanistic burden of Pompe disease suggests that beyond an initial improvement on first introduction of ERT, most patients have a quality of life that remains well below typical population norms, with certain symptoms, such as pain, being largely unaffected by ERT [ 19 – 25 ].
The overall economic burden of a chronic, progressive disease such as Pompe disease is established by evaluating the various components that contribute to the total cost of the disease. These components include direct medical costs (such as costs of treatment and medical devices), direct non-medical costs (including care in the home and transport), and indirect costs (including the costs of informal care, predominantly from parents, spouses and other family members, and productivity losses). Only when all of these elements are considered in total can the true economic burden of the condition to society be established.
Literature reviews have previously been published on the clinical or humanistic burden of Pompe disease [ 6 – 8 , 25 , 26 ]. However, relatively little attention has been paid to economic outcomes of the disease. The objectives of this systematic review were to characterize the health economic evidence on Pompe disease, including healthcare resource use and costs (direct and indirect), health utilities, and the cost-effectiveness of current treatments (incremental cost per quality-adjusted life year [QALY] gained) used to manage IOPD and LOPD patients.
No ethical approval was required because this study was a systematic review. A Preferred Reporting Items for Systematic Reviews and Meta-Analyses (PRISMA) checklist [ 27 ] and review protocol summary are provided as Electronic Supplementary Material.
Search Strategy
This review involved systematic searches in Embase ® /MEDLINE ® using Embase.com ® to identify relevant articles published through end of December 2017 on the economic burden of Pompe disease. Each search was conducted using controlled vocabulary and key words and was limited to articles published in English and studies involving human subjects. The specific search terms used are provided as Electronic Supplementary Material. Search terms were selected to ensure a broad coverage of evidence types given the limited literature in the space and to identify economic evaluations that incorporated effectiveness evidence. To supplement the systematic searches, additional papers were identified through bibliography reviews of relevant articles and other web-based sources.
Selection Criteria
Titles and abstracts of articles identified were carefully screened by a single reviewer (DG) in the initial review for relevance to the topic. Articles were selected for inclusion based on predefined acceptance criteria, which included patient population (adults/children diagnosed with Pompe disease), outcome measures of interest (cost, healthcare resource use, health utilities, cost-effectiveness) and study design (quantitative and/or qualitative data collection). Articles exclusively reporting clinical and/or humanistic outcomes were excluded as not relevant. Other exclusion criteria included non-English language and absence of peer review for articles, editorials, correspondence, and conference abstracts.
Articles identified as potentially relevant were obtained in full text for further evaluation. Every full-text article was screened, and its eligibility was confirmed by at least two reviewers. Inconsistencies were resolved through consensus.
Quality Assessment
A descriptive analysis of each publication was conducted during the data extraction stage. The reviewers assessed each publication for quality by considering the characteristics that could introduce bias. All studies were given a quality rating using the Newcastle–Ottawa Scale [ 28 ], which grades studies from 1 (poorest quality) to 9 (highest quality). Details of the grading awarded to the different studies are available in the Electronic Supplementary Material.
Data Extraction and Analysis
Data from the included studies were extracted into a predefined extraction grid by a single reviewer (DG), and included study design, setting, patient characteristics, outcome measures, key results and conclusions. Given the descriptive nature of this systematic review, extracted data were narratively synthesized and reviewed by all authors.
To present the currencies used in a standardized format, all data were inflation- and currency-adjusted to 2017 United States dollars (USD) to provide clarity when comparing across studies (see Electronic Supplementary Material).
Titles and abstracts of a total of 211 citations were screened following the initial searches, 16 of which were included for full-text screening. Eight articles were subsequently selected for final inclusion in this narrative synthesis (Fig. 1 ) [ 29 – 36 ]. Due to the substantial differences in patient characteristics and subsequent economic burden between IOPD and LOPD, these two phenotypes are considered separately in this review.

Flowchart of screening and identification process. IOPD infantile-onset Pompe disease, LOPD late-onset Pompe disease. a No meta-analysis was conducted in this review due to inadequate data
Two of the publications included were in IOPD patients only (in England/Colombia [ 29 ] and the Netherlands [ 30 ]), four were in patients with LOPD only (in the Netherlands [ 31 , 32 , 36 ] and Canada [ 33 ]), and two included both LOPD and IOPD patients (in the US [ 34 ] and England [ 35 ]). The two IOPD publications and one of the four LOPD publications were economic models that utilized data derived from published literature [ 29 ] or patient-level information that had not been previously presented [ 30 , 36 ]. The remaining publications were based on real-world data, mostly from two main registries: (1) the Dutch Erasmus Medical Center, an International Pompe Association (IPA) database; and (2) the National Collaborative Study of Lysosomal Storage Disorders, a multicenter, longitudinal, observational study in seven designated treatment centers in England.
Wyatt et al. presented cost data for children versus adults rather than for IOPD versus LOPD in England [ 35 ]. As it is not possible to separate the data according to phenotype, and as 85% of patients had LOPD, these data are described under the LOPD section (except for costs of ERT in IOPD, where data are presented for infants only). Data from the second mixed population study (conducted in the US) [ 34 ] are described in both the IOPD and LOPD discussions as appropriate.
An overview of the studies, including outcomes evaluated and quality ratings, is provided in Table 1 .
Table 1
Overview and outcomes evaluated for studies reporting on health economics of IOPD and LOPD
DRDWG Drugs for Rare Diseases Working Group, EQ - 5D EuroQoL-5D, ERT enzyme-replacement therapy, HCRU healthcare resource utilization, HRQoL health-related quality of life, IOPD infantile-onset Pompe disease, LOPD late-onset Pompe disease, NR not reported, QALY quality-adjusted life year, SF - 6D Short Form-6D
Health Economic Evidence of IOPD
Management of iopd is associated with a substantial economic burden.
In Pompe disease, the cost of purchasing and administering ERT dwarfs all other costs associated with the disease. As such, the costs of ERT are considered as a separate discussion. Here, we present costs of supportive care from an overall societal perspective from a single publication reporting a patient-simulation model based on data from 12 Dutch IOPD patients (average age at first measurement, 3.5 years) [ 30 ]. Using this model, total lifetime cost of an IOPD patient receiving supportive therapy only was €32,871 (currency and inflation-adjusted cost of $41,667); however, the average mean life expectancy of patients estimated using the economic model was just 0.40 years. No other data on the costs of supportive care in IOPD were available.
The Costs of Treating IOPD with ERT is Substantial
Data relating to the costs of ERT in IOPD are found in four publications, of which two are based on modeled data and two on real-world data. The modeled data include the Kanters et al. (2014) publication in a Dutch population described in the preceding section [ 30 ] and a second publication reporting a deterministic Markov model based on annual cycles using published literature from a health system perspective over a 20-year time horizon in England and Colombia [ 29 ]. Real-world data are taken from a retrospective analysis of the national Medicaid pharmacy claims database, which describes drug utilization and spending trends in the US Medicaid program [ 34 ] and a United Kingdom (UK)-based cohort study in which 62 out of 65 LOPD patients and all IOPD patients ( n = 12) received ERT [ 35 ]. In the latter study, the average time on ERT was 2.19 (range 0.15–9.7) years and 1.31 (range 0–3.12) years for IOPD and LOPD patients, respectively. This study reported cost data according to adult and child populations rather than by phenotype, and therefore cannot be separated into LOPD and IOPD patients.
Guo et al. [ 34 ] calculated US Medicaid quarterly prescriptions and reimbursement amounts for the first two ERTs available for Pompe disease: Myozyme and Lumizyme. Medicaid spending on Myozyme for IOPD was $3.6 million in 2010. Prescriptions for Myozyme increased from one in quarter 2, 2006, to 127 in quarter 2, 2011, with an average price per prescription of approximately $10,000. At $20,000, the authors also noted that the price per prescription for Lumizyme (indicated for LOPD in the US) was essentially double the price for Myozyme (indicated for IOPD). This difference was thought to be caused by the higher dosages required for larger LOPD patients compared with smaller infants with IOPD.
In terms of per-patient costs, Fig. 2 provides a summary of the IOPD ERT costs from both modeled data and real-world data in the Netherlands, England, Colombia, and US, including standardization as it relates to currency and inflation from the data year to 2017 (the individual studies corrected for the increasing weight of the child due to growth over time within their own methodology). There is wide variability across studies, with annual inflation- and currency-adjusted ERT costing from $37,132 for 20 mg/kg biweekly in the UK [ 35 ] to $207,604 for 20 mg/kg biweekly in the US [ 34 ] and $152,372 for 20 mg/kg biweekly in the Netherlands [ 30 ].

Annual total ERT costs per patient (IOPD; annual currency- and inflation-adjusted [2017 USD]) a . CPI consumer price index, ERT enzyme-replacement therapy, IOPD infantile-onset Pompe disease, OECD Organisation for Economic Cooperation and Development, USD United States dollars. a Standardized with respect to currency, costs, and dose (20 mg/kg/2 week). Total ERT costs represent drug acquisition cost only. These costs exclude infusion, supportive care, and other costs associated with ERT for Pompe disease. Annual cost is calculated based on total costs divided by total time alive for all studies except Guo et al. 2012. Annual costs were estimated based on costs and prescriptions dispensed per quarter (prescriptions adjusted to patients based on an assumed regimen of 20 mg/kg/2 weeks) to estimate an annual per-patient cost. Annual cost for 20 mg/kg every 2 weeks per patient calculated as €120,205 [ 32 ], £38,324 (Castro-Jaramillo 2012 – England), £41,678 (Castro-Jaramillo 2012—Colombia), $190,488 [ 36 ], and £26,025 [ 37 ]. Inflation-adjusted from data to year 2017 (inflation-adjusted to 2017 using average annual inflation rate per year reported by the OECD for each country and inflated based on data year) calculated as €135,215 [ 32 ], £44,109 (Castro-Jaramillo 2012—England), £50,013 (Castro-Jaramillo 2012—Colombia), $207,604 [ 36 ], and £28,852 [ 37 ]. OECD (2018), “Inflation consumer price index (CPI) (indicator).” 10.1787/eee82e6e-en (Accessed on 17 Jul 2018). Inflation-adjusted currency converted to USD based on currency reported in the publication. OECD (2018), “Exchange rates (indicator).” 10.1787/037ed317-en (Accessed on 17 Jul 2018)
Castro-Jaramillo modeled the cost-effectiveness of ERT (Myozyme, 20 mg/kg) given every 2 weeks to IOPD patients in England and Colombia [ 29 ]. Costs of the infusions and costs of managing complications from ERT application were included in the model. In this model, total annual costs per patient (average weight, 10 kg), including cost of ERT, were £194,342 (inflation- and currency-adjusted cost, $287,870) in England compared with £97,963 (adjusted, $166,418) in Colombia [ 29 ]. The largest component of cost in each case was use of the pediatric intensive care unit (£148,200 [adjusted, $219,522] and £49,351 [adjusted, $83,837] per patient for England and Colombia, respectively). ERT was the second largest cost component (£38,324 [adjusted, $56,768] and £41,678 [adjusted, $70,802] per patient for England and Colombia, respectively). The total lifetime cost from the cost-effectiveness analysis for the ERT arm was £1,337,118 (adjusted, $1,980,613) in England compared with £607,329 (adjusted, $1,031,719) in Colombia.
Kanters et al. (2014) modeled the total and incremental costs for ERT versus supportive care in IOPD in the Netherlands [ 30 ]. Total costs for patients treated with ERT were calculated based on four components: (1) cost of drug; (2) infusion-related costs; (3) costs related to other healthcare use; and (4) informal care costs. Patients receiving supportive therapy did not incur costs of the drug and infusion-related costs. Total lifetime cost was €7,032,899 (inflation- and currency-adjusted cost, $8,914,905) for ERT-treated patients, which represents an incremental lifetime cost of €7,000,028 (95% confidence interval [CI] 1,869,635–12,130,422) (adjusted, $8,873,238 [95% CI 2,369,950–15,376,527]) over supportive care alone. The majority (95%) of this was due to the specific costs of ERT and its infusion (€6,630,525 [adjusted, $8,404,856] and €212,793 [adjusted, $269,736], respectively). The increment for other costs (medical costs plus costs of informal care) was €156,711 (95% CI 131,728–181,694) (adjusted, $198,647 [95% CI 166,978–230,315]). It should be noted that the higher costs incurred by ERT-treated IOPD patients versus those treated with supportive therapy were not due solely to the ERT treatment, but also because they lived much longer (life expectancy of 13.79 years vs 0.4 years for the supportive treatment group).
Cost-Effectiveness of ERT in IOPD
The patient-simulation model described by Kanters et al. (2014) from the Netherlands also estimated the incremental costs per QALY at a dosage of 40 mg/kg/week (regimen used in some centers for IOPD) and 20 mg/kg biweekly (licensed dose) compared with supportive care alone [ 30 ]. At 40 mg/kg/week, the incremental cost per QALY was estimated to be €1.04 million (~ €7 million incremental costs and 6.75 incremental QALYs) and the incremental cost per life year gained was €0.5 million (~ €7 million incremental costs and 13.39 incremental life years); total costs were considerably lower in the 20 mg/kg biweekly analysis (Table 2 ). The incremental cost-effectiveness ratios (ICERs) at the 20 mg/kg biweekly dose were approximately 3.6 times lower (€286,114 [inflation- and currency-adjusted cost, $362,678]) than the base case ICER (40 mg/kg/week). The fact that physicians are increasingly preferring a 40 mg/kg biweekly, or even weekly, dosing regimen, adds considerably to the cost burden. However, it should be noted that clinical outcomes are generally improved at higher doses, creating the possibility of changes in QALY and/or life expectancy.
Table 2
Incremental cost-effectiveness ratios of ERT in IOPD including inflation and currency adjustment
CPI consumer price index, ERT enzyme-replacement therapy, GBP Great Britain pounds, ICER incremental cost-effectiveness ratio, IOPD infantile-onset Pompe disease, OECD Organisation for Economic Cooperation and Development, QALY quality-adjusted life year, USD United States dollars
a Inflation-adjusted from data to year 2017 (inflation-adjusted to 2017 using average annual inflation rate per year reported by the OECD for each country and inflated based on data year). OECD (2018), “Inflation consumer price index (CPI) (indicator).” 10.1787/eee82e6e-en (accessed on 17 July 2018). Inflation-adjusted currency converted to USD based on currency reported in the publication. OECD (2018), “Exchange rates (indicator).” 10.1787/037ed317-en (accessed on 17 July 2018)
As described in Sect. 3.2.2 , the cost-effectiveness of ERT (Myozyme, 20 mg/kg) given every 2 weeks to IOPD patients was modeled in England and Colombia [ 29 ]. In this study, the ICER per QALY gained was £234,308 (~ £1,187,940 incremental costs and 5.07 incremental QALYs) for England and £109,991 (~ £557,653 incremental costs and 5.07 incremental QALYs) for Colombia [ 29 ] (Table 2 ). The authors noted that ICERs per QALY were very high in both cases, compared with ‘established’ cost-effectiveness thresholds per QALY or year of life gained used in some other countries for decision making [ 37 ].
For instance, in England, National Institute for Health and Care Excellence (NICE) decisions appear to have been based on a cost-effectiveness threshold upwards of £20,000–£30,000/QALY [ 38 ]. In the US, the cost-effectiveness threshold can range from $100,000 to $150,000 for cancer drugs and $50,000 to $100,000 for noncancer drugs [ 39 ]. It should be noted that the time horizons for all three cost-effectiveness analyses were set to lifetime, but differences in discounting may have a significant impact on assessing results across different cost-effectiveness analyses. For example, Kanters et al. (2014) utilized recommendations set forth in the Dutch pharmacoeconomic guidelines assuming an annual discount of 4% for costs and 1.5% for effectiveness [ 30 ], while Castro-Jaramillo assumed 5% annual discounting for both costs and effectiveness when estimating ICERs for England and Columbia [ 29 ]. The resulting differences in incremental costs are due to differences in treatment and medical costs inputs between countries, while the differences in incremental QALYs reported (Kanters et al. [2014] 6.75 years vs 5.07 in Castro-Jaramillo) are the result of differences in health utility inputs and of discounting of effectiveness over the lifetime horizon.
Health Economic Evidence of LOPD
Lopd is associated with a substantial economic burden.
Data relating to the economic burden of LOPD (excluding ERT costs) are taken from two real-world publications; the first was a retrospective cohort study conducted in the Netherlands in 80 adult LOPD patients with mild-to-severe disease receiving supportive care only [ 31 ], and the second was the UK-based cohort study described previously [ 35 ]. The cost of ERT in LOPD is considered in the subsequent section.
Table 3 provides a summary of the costs reported in the two studies, including adjustment for inflation and currency. It is interesting to note that when the costs from the papers are standardized, there remains a substantial difference in overall annual costs (ranging from $8989 for an adult and $14,382 for a child in Wyatt et al. [ 35 ] to $28,489 in Kanters et al. [2011] [ 31 ]). This can largely be attributed to the absence of costs associated with informal care and productivity losses in the analysis by Wyatt et al. However, even comparing direct medical costs between the two studies, the standardized figure of $17,340 calculated from Kanters et al. (2011) [ 31 ] exceeds the corresponding value of $14,301 for adults, and is considerably higher than the figure of $6422 calculated for children from the Wyatt study [ 35 ]. These differences are purely due to differences in the cost of care, and are not explained by the type of service, currency, or data year. As well as the obvious differences in treatment costs between adults and children, these differences may be explained by the fact that the inflation- and currency-adjusted figures disregard differences in purchasing power and healthcare systems in different countries as well as inconsistencies in patient characteristics and reporting.
Table 3
Studies reporting supportive care costs in LOPD patients, including inflation and currency adjustment
CPI consumer price index, ED Emergency department, GBP Great Britain pounds, GP general practitioner, ICU intensive care unit, LOPD late-onset Pompe disease, OECD Organisation for Economic Cooperation and Development, USD United States dollars
a Inflation-adjusted from data to year 2017 (inflation-adjusted to 2017 using average annual inflation rate per year reported by OECD for each country and inflated based on data year). OECD (2018), “Inflation consumer price index (CPI) (indicator).” 10.1787/eee82e6e-en (accessed on 17 July 2018). Inflation-adjusted currency converted to USD based on currency reported in the publication. OECD (2018), “Exchange rates (indicator).” 10.1787/037ed317-en (Accessed on 17 July 2018)
b Individual reported mean annual costs for Kanters et al. (2011) [ 31 ] do not exactly sum to the overall cost due to rounding
Total estimated inflation- and currency-adjusted costs for each LOPD patient receiving supportive care in the Netherlands were $28,489 per year (range, $0–$214,908) [ 31 ]. Direct medical costs (defined as hospital days, intensive care, nursing home, ambulatory care, medication and other medical costs including tests, procedures, respiratory support, and medical devices) accounted for the majority (61%) of the total estimated costs ($17,340 per patient per year) in LOPD. Non-medical direct costs (transportation and other non-medical care) accounted for 2% of the total cost ($534), while indirect costs (informal care and productivity losses) totaled $7838 (37%). In terms of healthcare resources used by adult LOPD patients receiving supportive care, Kanters et al. (2011) reported that patients averaged two visits to the general practitioner (GP) per year and five outpatient hospital visits, most commonly to a neurologist (28%) [ 31 ]. In summary, almost half of the estimated mean annual total health and social care cost per adult (excluding ERT) was attributed to NHS hospital services ($4280 of total cost of $8989), while among children, NHS hospital services accounted for 92% of total health and social-care costs ($13,269 of total cost of $14,382) [ 35 ].
As well as direct costs of care, indirect economic costs must also be considered in relation to the overall economic burden of a disease. Indirect economic burden has two major components: costs of informal care (predominantly from spouses and other family members) and productivity losses. Kanters et al. (2011) reported that 85% of patients in the Netherlands received informal care, usually from more than one caregiver, suggesting a significant impact on the patient’s social network [ 31 ]. The total cost of the informal care was estimated at €5741 annually (range, €0–36,037) (inflation- and currency-adjusted cost, $7277 [range, $0–45,681]), accounting for 26% of the total annual costs of €22,475.
In terms of productivity losses, Kanters et al. (2011) reported that only 31 of 80 patients (39%) were employed, with 32 patients (40%) indicating that they had stopped working due to Pompe disease [ 31 ]. Of those patients who were working, 32% were reported absent from work due to illness for an average of 12 work days per year. Productivity losses were estimated at €2633 (range, €0–38,176) per patient per year (inflation- and currency-adjusted cost, $3338 [range, $0–48,392]), which accounted for 12% of total annual disease-related costs [ 31 ].
Costs of ERT in LOPD are Substantial
Real-world data on the costs of ERT in LOPD are taken from four studies conducted in the US [ 34 ], England [ 35 ], Canada [ 33 ], and the Netherlands [ 36 ]. Figure 3 provides a summary of these cost studies, including standardization across identified studies as it related to the currency and inflation from the data year to 2017. The data were relatively homogeneous, with adjusted annual costs of ERT for 20 mg/kg biweekly ranging from $503,118 [ 33 ] to $547,835 [ 34 ] for patients classified as having LOPD, and $173,753 to $403,490 for patients classified as children or adults, respectively [ 35 ]. Overall lifetime costs for LOPD were not reported in any of the publications.

Annual total ERT costs per patient (LOPD; annual currency- and inflation-adjusted [2017 USD]) a . CAN$ Canadian dollar, ERT enzyme-replacement therapy, LOPD late-onset Pompe disease, OECD Organisation for Economic Cooperation and Development, USD United States dollars. a Total ERT costs represent drug acquisition cost only. These costs exclude infusion, supportive care, and other costs associated with ERT for Pompe disease. Annual cost is calculated based on total costs divided by total time alive for Wyatt et al. 2012. Annual costs for Guo et al. 2012 were estimated based on costs and prescriptions dispensed per quarter (prescriptions adjusted to patients based on an assumed regimen of 20 mg/kg/2 weeks) to estimate an annual per patient cost. Annual costs were reported directly from Winquist et al. 2014. Annual cost for 20 mg/kg every 2 weeks per patient calculated as $502,667 [ 36 ], £282,798 (adult [ 37 ]), £121,780 (child [ 37 ]), and CAN$600,000 [ 35 ]. Inflation-adjusted from data to year 2017 (inflation-adjusted to 2017 using average annual inflation rate per year reported by the OECD for each country and inflated based on data year) calculated as $547,835 [ 36 ], £313,513 (adult [ 37 ]), £135,007 (child [ 37 ]), and CAN$652,915 [ 35 ]. Inflation-adjusted currency converted to USD based on currency reported in the publication. OECD (2018), “Exchange rates (indicator).” 10.1787/037ed317-en (accessed on 17 July 2018)
Winquist et al. [ 33 ] conducted a retrospective observational cohort study as part of a Canadian policy framework intended to evaluate the public funding of drugs for rare diseases. They reported the cost of ERT as $840 per 50-mg vial, which was calculated to be equivalent to $600,000 annually for an LOPD patient weighing 70 kg, leading to a total drug expenditure of $16,861,408 between June 2009 and June 2012.
As described previously, Wyatt et al. [ 35 ] reported the annual cost of caring for people with Pompe disease in England, excluding the purchase cost of ERT, at an estimated £6300 for an adult and £10,080 for a child. In comparison, the mean annual cost of ERT was substantially greater at £282,798 for adults and £121,780 for children with Pompe disease. These findings confirm that the cost of the ERT itself dominates all other costs associated with LOPD.
Guo et al. reported that Lumizyme prescriptions in the US increased from six in quarter 3, 2010, to 60 in quarter 2, 2011, and expenditures rose from $119,691 to $1.16 million during the same period [ 34 ]. The average price per prescription was approximately $20,000, and in the first two quarters of 2011, Medicaid spending for Lumizyme totaled $1.8 million [ 34 ].
Patients with LOPD Tend to Have Low Health Utilities Versus the Normal Population
Real-world data on health utilities among LOPD patients measured using the EuroQoL-5D (EQ-5D) (version not specified) or Short Form-6D (SF-6D) scales are taken from Kanters et al. (2011) [ 31 ] (Dutch patients receiving only supportive care) and Kanters et al. (2015) [ 32 ] (Dutch patients treated with ERT) using Dutch tariffs.
Kanters et al. (2011) reported an overall health utility score of 0.72 (standard deviation [SD], 0.18; range, 0.17–1.00) among untreated LOPD patients, as measured using the EQ-5D [ 31 ]. This is 17% lower than the utility score of the Dutch population as a whole (previously estimated at 0.87 [ 40 ]). At 0.15, the utility decrement arising from Pompe disease was statistically significant, and was ascribed predominantly to mild limitations in the domains of mobility, usual activities, and pain. The authors cautioned, however, that large variations in disease severity may undermine comparisons of health utilities between different diseases. In addition, patients with severe limitations were only observed in a small number of cases included in this analysis; as such, the impact on health utilities of Pompe disease from patients across the spectrum of disease severity may be underestimated.
Both EQ-5D and SF-6D among LOPD patients receiving ERT were evaluated by Kanters et al. (2015) [ 32 ]. Mean (SD) EQ-5D and SF-6D utility scores were reported to be 0.670 (0.201) and 0.699 (0.092), respectively. The authors reported that the maximum utility score of 1 was achieved in 6% of the EQ-5D observations, but was not observed in any patients on the SF-6D scale. In addition, the lowest possible score (− 0.35 for EQ-5D and 0.291 for SF-6D) was not observed in either instrument among these patients.
Cost-Effectiveness of ERT in LOPD
Data on the cost-effectiveness of ERT can be drawn from a simulation model using Dutch patient-level data from a societal perspective over a lifetime horizon published by Kanters et al. (2017) [ 36 ]. This study modeled two scenarios: a conservative approach that assumed no effects of ERT on survival after the observed period (scenario 1) and a projected approach in which the effect of ERT on survival was extrapolated beyond the observation period by carrying forward the estimated treatment-specific survival probabilities at the end of the observation period (scenario 2). In this analysis, the ICERs were lower in scenario 2 (€1.4 million [inflation- and currency-adjusted, $1,602,570] per life year gained and €1.8 million [adjusted, $2,045,855] per incremental QALY for scenario 2 vs €3.4 million [adjusted, $3,940,590]) per life year gained and €3.2 [adjusted, $3,652,575] per incremental QALY for scenario 1).
Pompe disease can have devastating consequences for both patients and their families. The clinical and humanistic burden of Pompe disease has been reviewed elsewhere [ 4 – 8 , 25 , 26 ]; however, to date, no systematic review on the health economic evidence of Pompe disease has been published. Here, we present the findings from eight published papers, and describe systematically the economic burden in both LOPD and IOPD.
As described elsewhere, the impact of Pompe disease on quality of life is substantial. Within an economic framework, this review shows the overall utility score to range from 0.67 to 0.72 [ 31 , 32 ]. This compares with an average utility score of 0.87 in the Dutch population as a whole, showing that Pompe disease patients have a utility deficit of > 0.1 on a scale that is commonly (not always) scored from 0 to 1, and represents a potentially clinically meaningful preference to avoid the humanistic burden associated with this disease.
The data included here confirm the substantial economic burden associated with both LOPD and IOPD, despite the relatively small numbers of patients affected. In terms of IOPD, the total lifetime cost of receiving supportive therapy only was estimated to be €32,871 ($41,667) [ 30 ], over a life expectancy of 0.4 years. Among adult LOPD patients receiving supportive care only, the average annual total cost for each patient was reported to be €22,475 (currency- and inflation-adjusted [2017] equivalent; $28,489) [ 31 ]. The largest cost components in LOPD among patients receiving supportive care were home care (31%), informal care (26%), and productivity losses (12%).
Resource use among LOPD patients was generally high, including both inpatient and outpatient visits, physicians, physical therapy, occupational therapists, social workers, nursing homes, ambulation devices, and respiratory support. Nursing home admissions accounted for 19% of the total €13,679 annual medical costs, while respiratory support accounted for only €574 per patient annually [ 31 ]. It is important to note that LOPD is, phenotypically, extremely heterogeneous, with ages of onset ranging from 12 months to late adulthood. Patients who have survived to adulthood without receiving treatment are likely to be those on the milder end of the spectrum and, as such, may require less care and medical intervention than those patients with more severe forms of the disease. These results should, therefore, be interpreted with caution when applying them to the Pompe disease population as a whole.
Despite these caveats, it was reported that among LOPD patients treated with ERT, the estimated mean annual cost of caring for patients, excluding the costs of ERT, was only £6300 ($8989) [ 35 ]. This is substantially less than the €22,475 ($28,489) reported by Kanters et al. (2011) [ 31 ], although Wyatt et al. did not include costs associated with informal care and productivity losses, which were the two major components in the latter publication.
Of note, this review also reveals that costs and resource use were significantly higher and health utilities were lower for both ERT-treated and non-treated LOPD patients using ambulatory or respiratory devices compared with patients not requiring these devices [ 31 , 32 ]. This finding is not surprising given the greater costs associated with wheelchair use and respiratory devices in other conditions [ 41 , 42 ] and the fact that their use indicates greater disease severity and progression. Furthermore, utility scores were lower among LOPD patients requiring ambulatory support compared with those using ventilator support, suggesting that the need for ambulatory support has a greater impact on the ability to lead a normal life and that these patients may require more caregiver support.
In terms of indirect costs, Kanters et al. (2011) reported that 85% of patients received informal care, and also found a considerable impact of Pompe disease on productivity [ 31 ]. In their study of untreated adult patients with LOPD, only 39% of patients were employed, with 40% indicating that they had stopped working due to Pompe disease. Of those who worked, 32% were reported absent from work due to illness for an average of 12 work days per year, 52% said they worked an average of 14 h a week less than they would without Pompe disease, and 19% would have applied for a job at a higher functional level if they had not been affected by the disease. Productivity losses due to Pompe disease were estimated to account for 12% of total annual disease-related costs.
Finally, the costs of treating Pompe disease patients with ERT are vast (Figs. 2 and and3). 3 ). For example, at £6300 ($8989), the annual cost of caring for adults with LOPD in the UK (excluding the purchase cost of ERT) is dwarfed by the mean annual cost of ERT, estimated at £282,798 ($403,490) [ 35 ]. This was consistent across studies and countries, with adjusted annual costs of ERT for adults estimated at $503,118 (Canada [ 33 ]) and $547,835 (US [ 34 ]). In IOPD, the lifetime incremental costs associated with ERT use are estimated to be €7.0 million ($8,873,238), with the majority (95%) of the incremental costs due to ERT itself, followed by infusion costs [ 30 ]. Since some IOPD patients are now treated with higher doses (40 mg/kg biweekly or even weekly) than initially recommended (20 mg/kg biweekly) [ 43 ], this adds considerably to the cost burden. It is important to note, however, that the considerable incremental lifetime costs in ERT-treated IOPD patients are not simply due to the ERT treatment itself, but also because of the substantially longer life expectancy of ERT-treated patients (around 14 years) compared with those managed with supportive care only (a few months only) [ 30 ].
ICERs, defined as the incremental costs per QALY gained at the 20 mg/kg biweekly dose, ranged from £109,991 ($186,851) for Colombia [ 29 ] to £234,308 ($347,070) for England [ 29 ] and €286,114 ($362,678) for the Netherlands [ 30 ]. At a dosage of 40 mg/kg/week, the ICER was €1,043,868 ($1,323,207) [ 30 ]. Thus, while there are large differences reported across different countries and healthcare systems, the ICER for ERT in IOPD is consistently high when compared with some established cost-effectiveness thresholds.
Gaps and Limitations of this Review
Despite a full systematic literature search, only eight publications were found reporting on the health economic evidence of Pompe disease (four in LOPD, two in IOPD and two in both LOPD and IOPD). The most robust evidence for IOPD, from two of four publications, is focused on cost-effectiveness evaluations [ 29 , 34 ]. The two other studies that included IOPD patients in the US [ 34 ] and England [ 35 ] specifically focus on drug costs only for ERT. The US study only focuses on the total budget impact, but calculated per prescription and not per patient cost. In terms of LOPD data, three of the six main studies come from the same source data (the IPA/Erasmus Medical Center Pompe Survey) [ 31 , 32 , 36 ], and include both analyses of ERT and non-ERT treatment populations as well as combined evaluations. A fourth LOPD study also focuses solely on drug costs per patient and lacks transparency, in that it is the application of a policy framework for rare disease therapy coverage decisions. Furthermore, that study focused only on cost per patient and budget impact [ 33 ].
This review reveals a substantial gap in health economic evidence relating to Pompe disease in countries beyond the Netherlands and England. In addition, the available evidence is mixed, as many of the cost studies specifically exclude a cost-effectiveness analysis due to the paucity of data and the expectation that cost-effectiveness analysis ratios would be extremely high and not informative. Indeed, where cost-effectiveness analyses were performed by Kanters et al. in multiple publications, the models do produce ICERs well above the willingness-to-pay thresholds. It should be noted that disregarding discounting in cost-effectiveness analyses may result in an underestimation of treatment costs, particularly given the lifetime time horizon. Furthermore, the models used vary in assumptions and inputs, requiring caution when comparing results across models and countries. The models are primarily driven by the ERT costs, and assumptions of the impact of ERT on survival vary among models, affecting health utilities adjustments for ERT versus non-ERT-treated patients. Although additional information may be available on the costs and cost-effectiveness in reimbursement decisions around the world, these sources fall outside the inclusion criteria for our review as they are largely non-peer reviewed. As described in Sect. 2.2, our review was restricted to peer-reviewed articles describing cohort, cross-sectional, case–control studies and randomized controlled trials.
For IOPD, there is a complete absence of published data on the indirect economic burden, health utilities and clinical drivers of cost and resource use, meaning that it was not possible to create a complete picture of the economic burden of IOPD. To partially address this gap, further insights can be taken from the published literature on a comparable disease such as spinal muscular atrophy (SMA). For example, one 2016 study reported an average cost of illness of SMA patients in Germany of €70,566/year per patient, consisting of €14,342/year per patient in direct medical costs (20% of the overall cost of illness), €40,378/year in direct non-medical costs (58%) and €15,845/year in total indirect costs (22%) [ 44 ].
For LOPD, data on untreated patients receiving supportive care only are drawn from a single study, and for those treated with ERT, only one study was entirely prospective, meaning that there were limitations to the information that was available and the conclusions that could be drawn. There were no data on indirect economic burden in LOPD in the post-ERT setting, while there were only limited data correlating disease progression with poor health utilities and higher costs or resource use following ERT. Additionally, due to the small sample size, no meta-analysis was possible. As such, there are limitations to the conclusions that can be drawn from this systematic review.
Other limitations derive from the nature of the studies reported. Most of the studies included have been rated on the Newcastle–Ottawa Scale as being of relatively poor quality (scoring 2–5 out of a maximum of 9). The low scores reflect deficiencies in the studies in terms of selection of cohorts, comparability of cohorts, and assessment of outcome. For example, patients who had survived for any length of time on supportive care only are likely to have been on the mild end of the disease spectrum, and may not be truly reflective of the wider patient population. Similarly, milder patients are less likely to receive respiratory or ambulatory support, and thus including them in analyses of healthcare resource use may underrepresent the total cost for the overall patient population. Another consideration is the impact of the weight of patients (particularly children, which may vary widely) on treatment costs, such that each study that uses different weights will have different treatment costs per patient. Data were adjusted to a standard dosing and frequency factor, but this adjustment does not take into account patient weight. Therefore, the average cost of a pediatric patient will be much lower than that of an adult, despite the same dosing regimen.
Like most other systematic reviews of the literature, this review suffers from potential publication bias. In general, this bias exists because studies that report positive associations are more likely to be published. Therefore, it is possible that studies containing valuable data may have gone undetected. Since we restricted this systematic review to studies published in English, it is possible that language bias may have affected our conclusions. Despite these limitations, we believe that the available literature reviewed here demonstrates the paucity of data in this area and the substantial cost associated with Pompe disease.
Avenues for Future Research
This systematic review highlights the relative lack of consistent, validated data evaluating the health economics of Pompe disease. As new therapeutic approaches become available, it will be increasingly important, in addition to the clinical relevance of a new drug, to accurately assess the true economic burden and health economic value of treating both LOPD and IOPD.
One important avenue of research will be to consider how clinical progression impacts the economic burden of Pompe disease in terms of costs, resource use, and health utilities. Previous studies have demonstrated continued disease progression despite ERT therapy [ 19 – 21 , 45 , 46 ], and it is therefore important that this effect be captured in future economic models. Another important research question that has not been addressed to date relates to the impact of ERT on the indirect economic burden and health utilities in Pompe disease. Until a quantitative evaluation of the change in indirect economic burden and health utilities following the advent of ERT for the treatment of Pompe disease is realized, our understanding of the overall economic burden of ERT-treated patients will remain incomplete.
Conclusions
Here, we present the first systematic review of the health economics of Pompe disease. This adds to other literature describing the significant clinical and humanistic burden Pompe disease presents. While there remain substantial gaps in the published literature, available data demonstrate that there is a high, persistent cost to patients, families, healthcare systems, and society in the presence and absence of ERT. Further research is needed to fully understand the complete picture across the spectrum of the disease, but it is clear that there remains a substantial cost associated with Pompe disease that is not fully addressed with existing treatment paradigms.
Below is the link to the electronic supplementary material.
Acknowledgments
The authors would like to thank Rachel Danks for assistance with the preparation of this manuscript.
Availability of Data and Materials
Authors’ contributions.
BS, EJ and SP contributed to the conception and design of the study. DG designed and carried out the search strategies. All authors were responsible for acquisition, analysis and interpretation of data. All authors drafted the manuscript. All authors read, critically revised and approved the final manuscript.
Compliance with Ethical Standards
The literature review and editorial assistance for the manuscript was funded by Audentes Therapeutics.
BS is a member of the Audentes Therapeutics Board of Scientific and Clinical Advisors. BS has received unrestricted research support, honoraria, and travel funding from Sanofi Genzyme during the past 5 years. BS received honoraria and travel funding as a member of the Global Advisory Boards from Biomarin Pharmaceutical, Lupin therapeutics, Nexien biopharm, Amicus Therapeutics, and Audentes Therapeutics. EJ and SP are employed by and own stock in Audentes Therapeutics, DG is a paid consultant of Audentes Therapeutics, and MG is an employee of BluePath Solutions, a company that receives consulting fees from Audentes Therapeutics. AH has no conflicts of interest.
Not applicable.
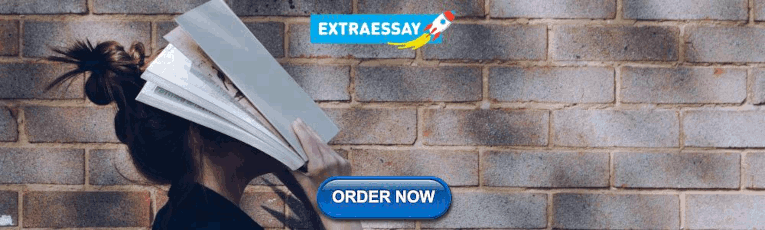
IMAGES
VIDEO
COMMENTS
Pompe disease is a genetic glycogen storage disorder with an autosomal recessive pattern of inheritance.1,2 The epidemiology of the disease is not clearly ... In this paper, we review the evidence published on ... This figure shows the different available/under research treatment strategies for Pompe disease. RhGAA's uptake is mediated by CI ...
The diagnosis of Pompe disease is ultimately confirmed with enzyme assays and genetic testing. Clinical history, exam, muscle enzymes, and electromyography (EMG) are the core of the initial workup and are used to help determine which patient should undergo further testing specific to Pompe disease, particularly in the setting of LOPD [].A clinical history of a limb-girdle pattern of weakness ...
Disease-specific therapy is available for only a handful of neuromuscular disorders, including Pompe disease—a debilitating metabolic myopathy caused by a deficiency of the lysosomal glycogen-catabolising enzyme, acid α-glucosidase (GAA). Enzyme replacement therapy (ERT) with recombinant human GAA, alglucosidase alfa, was approved (by the US Food and Drug Administration and the European ...
Pompe disease (PD) is an autosomal recessive disorder caused by mutations in the GAA gene that lead to a deficiency in the acid alpha-glucosidase enzyme. ... provides an outlook for future research directions and describes possible research applications. Feature papers are submitted upon individual invitation or recommendation by the scientific ...
Pompe disease (PD) is an inherited metabolic disorder caused by a deficiency of acid α-glucosidase (GAA), leading to lysosomal accumulation of glycogen, mainly in skeletal and cardiac muscles as well as the nervous system. ... Feature papers represent the most advanced research with significant potential for high impact in the field. A Feature ...
Pompe disease is a rare inherited lisosomal storage disease characterized by accumulation of glycogen in muscular cells due to a deficient activity of acid a-glucosidase (GAA), an enzyme which ...
Pompe disease, also known as glycogen storage disease type II, is caused by the lack or deficiency of a single enzyme, lysosomal acid alpha-glucosidase, leading to severe cardiac and skeletal muscle myopathy due to progressive accumulation of glycogen. ... Feature papers represent the most advanced research with significant potential for high ...
A research paper is a document that presents the findings of a scientific study or research project. It is written by researchers who conduct experiments or investigations to explore a specific topic or question. The paper follows a specific format, including sections like introduction, methods, results, discussion, and conclusion.
Pompe's disease, glycogen-storage disease type II, and acid maltase deficiency are alternative names for the same metabolic disorder. It is a pan-ethnic autosomal recessive trait characterised by acid α-glucosidase deficiency leading to lysosomal glycogen storage. Pompe's disease is also regarded as a muscular disorder, but the generalised storage of glycogen causes more than mobility and ...
Research paper. Gene therapy with secreted acid alpha-glucosidase rescues Pompe disease in a novel mouse model with early-onset spinal cord and respiratory defects. ... Pompe disease (PD) or glycogen storage disease type II (GSDII, OMIM # 232300) is a rare (~1 in 40,000 births) ...
Background and History. Pompe disease, a severe metabolic myopathy, is caused by mutations in the gene coding for acid alpha-glucosidase (GAA), the enzyme that breaks down glycogen in acidic milieu of the lysosome. Once in the lysosome, glycogen can escape following complete degradation by GAA in the form of glucose.
Pompe disease is a rare and deadly muscle disorder. As a clinical entity, the disease has been known for over 75 years. While an optimist might be excited about the advances made during this time, a pessimist would note that we have yet to find a cure. However, both sides would agree that many findings in basic science—such as the Nobel prize-winning discoveries of glycogen metabolism, the ...
In the USA, one person in every 10,000 to 28,000 people is born with Pompe disease. Pompe disease develops when both parents pass on a copy of a gene, called GAA ... Arnold Reuser and Priya Kishnani were authors on the original paper and were involved in preparing this summary. ... Register to receive personalised research and resources by ...
How past discoveries of glycogen metabolism, the lysosome, and autophagy have guided Pompe research and impacted recent therapeutic developments is discussed. Pompe disease is a rare and deadly muscle disorder. As a clinical entity, the disease has been known for over 75 years. While an optimist might be excited about the advances made during this time, a pessimist would note that we have yet ...
The Lantern Project is an ongoing complimentary diagnostic program for patients in the United States sponsored by Sanofi and implemented by PerkinElmer Genomics. It combines specific enzymatic, biomarker, and genetic testing to facilitate rapid, accurate laboratory diagnosis of Pompe disease and sev …
Pompe disease is also considered a polyglucosan vacuolar myopathy which results from ... (8%). 15 Also, as newborn screening for metabolic disorders utilizes blood collected on dried filter papers (DBS), various platforms to measure GAA activity in DBS ... King Faisal Specialist Hospital & Research Center, MBC-03-30. PO Box 3354. Riyadh, 11211. ...
Chemical Science, 2021; DOI: 10.1039/D1SC03188K. University of Maryland. "Study could offer hope to Pompe disease patients." ScienceDaily. ScienceDaily, 19 August 2021. <www.sciencedaily.com ...
The clinical and humanistic burden of Pompe disease has been reviewed elsewhere [4-8, 25, 26]; however, to date, no systematic review on the health economic evidence of Pompe disease has been published. Here, we present the findings from eight published papers, and describe systematically the economic burden in both LOPD and IOPD.